Bahar Shahidi, James C. Hubbard, Richard L. Lieber and Samuel R. Ward
INTRODUCTION
Spinal muscles in coordination with the rest of the neuromusculoskeletal system (i.e., vertebrae, tendons, ligaments, and the nervous system), provide stability needed to protect vital anatomic structures and, along with the spinal column and neural control, have been described as one of three subsystems that must work together to stabilize the spine.1 Spinal musculature dysfunction is hypothesized to play a role in a variety of pathologic conditions, such as segmental instability, low back pain, and degenerative disc syndromes. However, the specific mechanisms that relate muscle function (or dysfunction) to pathologic processes are unclear. Some factors that lead to pathologic processes may be elucidated by biomechanical analyses of spine kinematics along with the associated tissue strains and loads. Such analyses rely heavily on accurate knowledge of muscle forces, moment arms, and activation patterns, which is frequently unavailable for spinal muscles, to calculate loads and displacements. Often, spinal muscles are ignored or overly simplified (e.g., lumped together as one muscle) because the anatomy of these muscles is considered too complex to represent realistically. However, the complex anatomy, architecture, and quality of spinal muscles profoundly influence their function. Thus, this information must be incorporated into analyses to accurately predict the role of these muscles in spinal function and dysfunction.
THE EFFECTS OF MUSCLE ARCHITECTURE ON FUNCTION
Functional properties of muscles are largely determined by their architecture—the number and arrangement of muscle fibers about the axis of force generation.2,3,4 Whereas muscle fiber diameter is relatively consistent among muscles of different sizes, architectural differences between muscles are much more variable and provide the basis for the specialized function of individual muscles throughout the body. Thus, muscle architecture is a primary determinant of muscle function, and understanding this structure-function relationship is of great practical importance, not only in clarifying the physiologic basis for production of force and movement, but also in providing a scientific rationale for surgery or rehabilitation. Muscle architectural studies also guide electrode placement for electromyographic (EMG) measures of muscle activity, explain the mechanical basis of muscle injury during movement, and aid in the interpretation of muscle quality through histologic specimens obtained from muscle biopsies.
Experimental Determination of Skeletal Muscle Architecture
Quantitative studies of muscle architecture were pioneered by Gans et al.,4,5 who developed a precise methodology for defining muscle architecture based on microdissection of whole muscles. The parameters usually included in an architectural analysis are pennation angle (i.e., the fiber angle relative to the axis of force-generation), muscle length, fiber or fascicle length, and physiologic cross-sectional area (PCSA) (Fig. 9-1). Pennation angle (q) is measured by determining the average angle of the fibers relative to the axis of force generation. Variation in pennation between fibers across a single muscle has been documented in several spinal muscles. However, variations in pennation angle do not seem to dramatically affect function.4 Muscle length is defined as “the distance from the origin of the most proximal muscle fibers to the insertion of the most distal fibers.”6 Fiber length represents the number of sarcomeres in series, and experimental evidence suggests that muscle fiber length is proportional to muscle excursion with contraction, as well as velocity of muscle contraction.5,7 Muscle fiber length can only be determined by microdissection of individual fibers from fixed tissues or by laborious identification of fibers by glycogen depletion followed by serial sections along the length of the muscle.8 Unless investigators are explicit when they refer to muscle fiber length, they are probably referring to muscle fascicle length (also known as fiber bundle length) because it is extremely difficult to isolate intact fibers that run from origin to insertion, especially in mammalian tissue.8,9 Experimental studies of mammalian muscle suggest that individual muscle fibers do not extend the entire muscle length and may not even extend the entire length of a fascicle.8,9 Detailed studies of individual muscle fiber lengths have yet to be conducted in human spinal muscle. However, more sophisticated imaging techniques (e.g. diffusion tensor imaging) are in development that allows for the determination of single fiber bundle lengths and pennation angles within a whole muscle and are providing fantastic new views of the complexity of spinal muscle fiber arrangement. However, it is important to note that these imaging approaches provide lengths that are not normalized fiber bundle lengths, so they cannot necessarily be used to predict muscle excursion or velocity, nor can they be used to calculate PCSA. In order to accurately interpret fiber bundle lengths, the final and critical experimental step required to perform architectural analysis of a whole muscle is to measure the average sarcomere length of isolated fibers. Because sarcomere lengths can vary during the fixation process, it is important to normalize fiber lengths to a constant, or optimal sarcomere length to ensure that a muscle fiber that measured as “long” or “short” was not merely fixed in a stretched or contracted position. Based on measured architectural parameters and joint properties, the relationship between sarcomere length and joint angle can be calculated. In addition, sarcomere lengths can be measured in live humans using intraoperative laser diffraction10 or less invasively with microendoscopy.11,12 Because sarcomere length strongly influences muscle force generation, an understanding of the relationship between sarcomere length change and movement has been used in many studies to provide added understanding of muscle design.10,13-16
The physiologic cross-sectional area (PCSA) is another critical architectural calculation. Theoretically, PCSA represents the sum of the cross-sectional areas of all the muscle fibers within the muscle, and thus it is the only architectural parameter that is directly proportional to the maximum tetanic tension generated by the muscle. The PCSA is almost never the same as the cross-sectional area of the muscle measured in any of the traditional anatomic planes, as would be obtained using a noninvasive imaging method such as MRI, CT, or ultrasonography. It is calculated as muscle volume divided by fiber length and has units of area. Because fibers may be oriented at a pennation angle relative to the axis of force generation, not all of the fiber tensile force is transmitted to the tendons. Specifically, if a muscle fiber is pulling with X units of force at a pennation angle (q) relative to the muscle axis of force generation, only a component of muscle fiber force (X • cosq) will actually be transmitted along the muscle axis. Thus, the volume/length is often multiplied by cosineq (pennation angle) to yield PCSA.
Anatomy and Architecture of Lumbar Spinal Musculature
The architecture of spinal muscles is complex and dramatically different from the architecture of appendicular muscles. For example, instead of distinct tendinous attachments to bone, many spinal muscles have very little tendon at their ends but have a complex arrangement of internal tendons and aponeuroses. Their attachments are broad, and many spinal muscles branch and have insertions at multiple vertebral levels. Some spinal muscles have short fascicles and high degrees of pennation, whereas others have long, parallel fascicles representing significant diversity in the function of the various muscle groups. All of these factors affect the force- and moment-generating capacity of muscles, which ultimately influence control of spinal movement and injury mechanisms.
Spinal muscles can be separated into two classes: intrinsic muscles, which connect vertebrae with each other; and extrinsic muscles, which attach vertebrae to the rib cage or pelvis. Embryologically, intrinsic muscles originate from the epimere, and extrinsic muscles originate from the hypomere. Intrinsic muscles receive innervation from the dorsal rami of spinal nerves and function to move and stabilize the vertebral column, whereas extrinsic muscles are innervated by the ventral rami of spinal nerves and help control the limb girdle or respiration.
Intrinsic Spinal Muscles of the Lumbar Spine
Intrinsic muscles of the spine are dominated by the erector spinae, a group of interdigitating muscles that spans the entire length of the spine from the base of the skull to the sacrum and iliac crest. Deeper than the erector spinae lies another important group of muscles, the smaller multifidus, which are described in more detail below. The erector spinae and multifidus muscles comprise the bulk of the thoracolumbar spinal musculature. These two distinct muscular units have large differences in innervation that probably result in significant functional differences,17 although the detailed biomechanical function of these groups remains only partially elucidated.18 The deepest muscles of the lumbar spine are the interspinales and intertransversarii; they originate and insert on the spinous and transverse processes of adjacent vertebrae, respectively. Additionally, the rotatores muscle originates from the lamina and inserts on the transverse process, one vertebral level caudal.
The erector spinae are commonly considered to be composed of three muscles; from medial to lateral, they are the spinalis, longissimus, and iliocostalis. Although there are varying definitions of the composition of the erector spinae, the study by MacIntosh and Bogduk18 provides the most comprehensive descriptive anatomy of the lumbar erector spinae to date, and Delp and colleagues19 provided the first architectural measurements of these muscles. These architectural properties include muscle tendon and fascicle length, sarcomere lengths, and PCSAs (see Table 9-1). Delp and colleagues found that fascicle lengths were approximately 30% of muscle lengths in the erector spinae muscles and that sarcomere lengths measured from cadavers in the supine position were generally shorter than the optimal length, which may imply that the erector spinae are capable of developing greater force in elongated positions (i.e., in flexion).20 This muscle may thus be designed to restore spine extension from flexion. Although longissimus and iliocostalis may have different functions because of their medial-to-lateral anatomical locations, their fiber type distributions are similar with approximately 60% type 1 fibers,21 the slowest muscle fiber type. The predominance of type 1 fibers implies that these muscles are relatively slow contracting and fatigue resistant compared to most appendicular muscles in which faster type 2 fibers tend to predominate. Functionally, this allows for these muscles to have more utility with tonic activation for postural control than for primary movement.22 It also implies that these muscles are activated a great deal of the time. The detailed anatomy of the erector spinae provided by MacIntosh and Bogduk18 also provides important information relevant to EMG studies. Because thoracic fascicles of the longissimus thoracis and iliocostalis lumborum lie superficially to lumbar fascicles, electrodes placed superficially at lumbar vertebral levels may not accurately represent the activity of fascicles directly attached to lumbar vertebrae.
Muscle | Musculotendon Length (cm) | Muscle Length (cm) | Fascicle Length (cm) | Pennation Angle (°) | Sarcomere Length (μm) | PCSA (cm2) |
---|---|---|---|---|---|---|
Rectus Abdominus | 35.9 (1.9) | 34.3 (2.7) | 28.3 (3.6) | 0.0 (0.0) | 2.83 (4.2) | 2.6 (0.9) |
Quadratus Lumborum (proximal) | 11.7 (1.7) | 10.7 (1.3) | 7.3 (1.3) | 7.4 (2.9) | 2.39 (0.21) | 1.6 (0.6) |
Quadratus Lumborum (distal) | 9.3 (1.3) | 8.1 (1.2) | 4.7 (0.5) | 7.4 (6.2) | 2.37 (0.20) | 1.2 (0.4) |
Spinalis Thoracis | 24.7 (1.5) | 18.2 (3.2) | 5.2 (0.4) | 16.0 (3.8) | 2.26 (0.17) | 1.6 (0.9) |
Longissimus Thoracis | 42.6 (5.5) | 34.7 (4.8) | 9.6 (1.2) | 12.6 (5.8) | 2.31 (0.17) | 5.9 (2.5) |
Iliocostalis Lumborum | 43.8 (4.3) | 33.1 (9.0) | 12.0 (1.7) | 13.8 (4.5) | 2.37 (0.17) | 4.1 (1.9) |
Multifidus | N/A | N/A | 4.8 (1.7) | 18.4 (4.2) | 2.26 (0.18) | 23.9 (8.4) |
The lumbar multifidus muscles consist of multiple distinct fasciculi originating from a single vertebral spinous process and lamina with individual bands inserting at the mamillary process adjacent to the superior facet from two to four segments below the level of origin. All multifidus bands arising from a given vertebral level are innervated by the medial branch of the primary dorsal ramus of the spinal nerve at that level. While the multifidus originates at a single level, and multiple distinct bands may insert and affect action at several different levels, each of these bands which share a common origin also shares a common innervation.23 This unisegmental innervation has implications for electromyography and diagnosis of zygapophysial joint pain related to abnormal activity in multifidus.
The principal action of the multifidus is to produce vertebral extension, but the multisegmental nature of the muscle, as well as the complex three-dimensional orientation in the cranial-caudal and mediolateral directions, renders this statement a gross oversimplification.24 The multifidus is not necessarily a primary mover of the spine; rather, its function is likely to produce small vertebral stabilizing movements. Similar to the erector spinae, the fiber type distribution of approximately 60% type 1 fibers seen in the multifidus supports this postural role.21,25
A recent study of the multifidus muscle revealed three major design factors that make it well-suited for stabilizing the lumbar spine.20 First, the architecture of the multifidus is highly pennated with fibers extending only approximately 20% of the length of the muscle. Thus, a large number of muscle fibers are contained in a small volume, and even though the multifidus has a smaller mass compared to several other lumbar extensors, it is predicted to create the greatest lumbar extension force by a factor of two. In fact, of all of the lumbar musculature, the multifidus has the largest PCSA and one of the shortest fiber lengths. This architecture is consistent with a muscle specialized for high-force, short-excursion movements, supporting its role as one of the most important stabilizers in the lumbar spine (Fig. 9-2). Second, the intraoperatively measured multifidus sarcomere length, is relatively short when the spine is extended, suggesting that the capacity of the muscle to generate force increases as it is stretched. In other words, as the spine flexes, multifidus force increases, suiting it to restore the vertebral spine toward neutral or more extended positions. Finally, direct mechanical testing comparing multifidus muscle fiber bundles to appendicular muscle has revealed that, while individual multifidus muscle fibers have the same mechanical properties as appendicular muscle fibers, whole multifidus bundle testing, which includes extracellular connective tissue, demonstrates a significantly higher elastic modulus. Thus, the multifidus as a whole muscle has a high passive elastic capacity, optimally suiting it for passive resistance to flexion of the lumbar spine.
Extrinsic Muscles Linking Vertebrae to the Pelvis
The quadratus lumborum attaches from the iliolumbar ligament and iliac crest onto the twelfth rib and transverse processes of L1 to L4. It assists in lateral bending of the lumbar spine. Electromyographic evidence shows that the quadratus lumborum has a dominant role in spine stabilization.26
The psoas major attaches from the anterior surface of the transverse processes, the lateral aspects of vertebral bodies, and intervertebral discs of all lumbar vertebrae. Together with the iliacus, which arises from the ilium, they form the iliopsoas that inserts on the lesser trochanter of the femur and is a major flexor of the thigh and trunk. The psoas is the largest muscle in cross section at the caudal levels of the lumbar spine.27 Biomechanical analysis shows that the psoas has the potential to laterally flex the lumbar spine, to generate compressive forces across vertebral levels that would increase lumbar spine stability, and to create large anterior shear forces at L5 to S1.28 However, if the psoas were designed for lumbar spine motions, one would expect an architectural design consisting of longer fascicles attaching to more rostral segments because they would undergo larger excursion. Instead, psoas fascicles generally have the same length regardless of level of origin, suggesting that the psoas is likely designed to flex the hip;4 EMG studies confirm that its primary function is hip flexion.29
Extrinsic Muscles Linking Vertebrae to the Rib Cage
The superior and inferior division of the serratus posterior attach the vertebral column to the ribs. The serratus posterior superior originates from the lower part of ligamentum nuchae and the spinous processes of the upper thoracic vertebrae and inserts on ribs two through five. The serratus posterior inferior originates from the spinous processes of the lower thoracic and upper lumbar vertebrae and inserts on ribs nine through twelve. These muscles function to elevate and depress the ribs, respectively.
The latissimus dorsi arises from the spinous processes of the lower six thoracic and upper two lumbar vertebrae, the thoracolumbar fascia, the iliac crest, and the lower ribs to insert on the humerus. The magnitudes of its potential force and moment on the lumbar spine and sacroiliac joint are small.30 It is generally considered to move the arm, but if the upper limb were fixed it has the potential to move the trunk (e.g., as in wheelchair transfers or crutch locomotion). However, a detailed biomechanical and anatomical study of the latissimus dorsi has yet to be performed.
To summarize, the spinal muscles are characterized by complex anatomy and architecture and have important biomechanical features as well as diversity in function that are revealed when the architecture is studied in detail. The architecture of many spinal muscles and the effects on function remain an open a rea for further research.
IMPLICATIONS OF SPINAL MUSCLE ANATOMY AND ARCHITECTURE FOR MOTOR CONTROL
Neural control of a muscle is directly related to the underlying architecture of the muscle. Although neural inputs can change the force generation and speed of muscle contraction, the effectiveness of a given neural input is altered by different muscle architectural features (e.g., PCSA and fiber length, respectively). In other words, nervous system commands are “interpreted” through the architectural features of muscles to control posture and movement. Understanding both the biomechanics and neural control of spinal muscles through models and experimental studies is vital to understanding their role in pain and injury mechanisms.
MOMENT ARM CHANGES WITH POSTURE
Different parts of a muscle may have different moment arms, and the magnitude and, in some cases, direction of these moment arms changes with posture. Furthermore, muscles that cross multiple joints (as most spinal muscles do) may have different mechanical functions at different joints. In the lumbar spine, posture changes the mechanical function of the erector spinae muscles. McGill et al.31 measured the fiber angles of longissimus thoracic and iliocostalis lumborum with the lumbar spine in neutral and full flexion using high-resolution ultrasound. They found that flexion changes the line of action of these muscles, decreasing their capacity to resist anterior shear forces and potentially increasing the risk of back injury.32
IMPLICATION OF SPINAL MUSCLE ANATOMY AND ARCHITECTURE FOR INJURY AND PAIN
There are at least three ways in which spinal muscles may be implicated in mechanisms of injury and back pain. First, the muscle itself may be injured through an eccentric contraction, which occurs when a muscle is rapidly lengthened while it is activated, leading to disruption of the muscular cytoskeleton within each of the fibers.33,34 This may occur during an imposed movement, particularly one in which the kinematics are abnormal. Second, muscle forces may alter the load distribution within anatomic structures that have been clinically linked to pain (e.g., the discoligamentous complex). Third, muscle activity can alter spinal stiffness and kinematics, which indirectly affects soft tissue loads and strains. The relationship between muscles and injury can be elucidated by biomechanical models, the validity of which depends on accurate modeling of anatomy and architecture.
MUSCLE ALTERING LOAD DISTRIBUTION IN OTHER ANATOMIC STRUCTURES
Because spinal muscles are oriented primarily vertically, their activation produces axial compression of the spine. The compressive loads on the discs and facet joints are a function of muscle force, moment arm, and activation. When the detailed anatomy of the lumbar erector spinae was included in a biomechanical model,35 the predicted disc compression and shear loads were reduced compared with a lumped extensor “muscle equivalent” commonly used in many models. This study highlights the importance of creating an accurate representation of muscle anatomy in biomechanical models. Compressive loading may severely alter surrounding tissue loads, particularly in the setting of abnormal vertebral kinematics. Muscles may also contribute to injury by overloading passive structures (e.g., bone, ligament, intervertebral disc). Conversely, if muscles are not appropriately activated during movement due to inhibition or deconditioning, the increased extrinsic load on the passive structures may increase the risk for injury and pain.36
MUSCLE EFFECTS ON SPINAL STIFFNESS AND STABILITY
It has long been recognized that muscles are necessary for spinal stability. However, it is unclear which muscles are most active during postural loads. This question has been addressed in several theoretical and experimental studies. Crisco and Panjabi37 examined the role of gross muscle anatomy (e.g., the number of joints crossed by a muscle) in the lateral stabilization of the lumbar spine using a mathematical model. They calculated minimal muscle stiffness necessary for spinal stability and found that muscles spanning only one vertebral body required the highest stiffness (i.e., activation) for stability, whereas those muscles that spanned the largest number of vertebrae were most efficient, requiring the least activation. Efficient stabilization (less muscle activation) is important because it implies lower disc loads. EMG-driven modeling by Cholewicki and McGill38 suggested that large muscles may provide the bulk of stiffness to the spinal column, as suggested by Crisco and Panjabi,37 but that the activity of short intrinsic muscles was also necessary to maintain stability. In fact, biomechanical models have shown that buckling (loss of stability) can occur from a temporary reduction in activation to one or more intersegmental muscles.38 Presumably, small intrinsic muscles are better suited to stabilize displacements at a single joint with a minimum increase in joint loads at other levels. However, despite the demonstration of the importance of both gross anatomy and architecture of spinal muscles on spinal stability in these models, many questions remain. Examples include the effect of muscle fatigue on spinal stability and the best muscle activation patterns for stability in the prevention and rehabilitation of low back pain.
Muscle fatigue has been implicated in low back pain;39 the mechanism may be related to altered loads in other structures, decreased spinal stability, accumulation of metabolites, or involvement of peripheral and central mediation of pain. In patients with pain as well as healthy subjects, fatigue can lead to dysfunction in neuromuscular control, including altered activation patterns or flexion-relaxation, which can increase passive tissue loading.40,41 There is some evidence for fiber type transformations from more fatigue-resistant slow-twitch type 1 toward readily fatigable fast-twitch type 2 in patients with back pain,42,43 but other studies have found that musculoskeletal disorders of the spine are not related to a change in fiber type.44,45 Fiber type studies in these muscles are extremely difficult due to their complex architecture and limited ability to perform muscle biopsies.
EFFECTS OF MUSCLE QUALITY ON FUNCTION
Although the average architectural features of the major muscles in the lumbar spine have been documented, there is an increasing need to generate patient-specific architectural data for diagnostic purposes, surgical planning, and musculoskeletal modeling. Further, recent investigations have demonstrated qualitative changes in muscle tissue to be an important feature in the lumbar spine and other joint systems subjected to chronic disease. Alterations in “muscle quality” have been demonstrated at both the macroscopic level through advanced imaging studies, as well as at the microscopic level through histological evaluation of biopsied tissue.
Recent advances in magnetic resonance imaging and other recently-developed image processing tools have allowed these muscles to be rapidly visualized and quantified in three dimensions in an unprecedented fashion (Fig. 9-3). Additionally, these tools can separate muscle tissue into contractile, fat, and even connective tissue compartments. As can be seen within a given muscle cross-sectional area (Fig. 9-4), the fraction of muscle tissue contained within “normal” muscle boundaries can vary significantly and may be substantially lower than anticipated. Recent work has demonstrated an association between paraspinal muscle fat fraction, but not paraspinal muscle CSA, and high-intensity pain and disability.46 Further, muscles with high levels of fatty infiltration seem to be resistant to exercise or other rehabilitative efforts47,48 and are also associated with recurrence and persistence of chronic low back pain as well as disc injury. Conversely, low levels of fatty infiltration in the lumbar multifidus muscles are associated with positive outcomes after surgery.48 Histologically, increased fat and connective tissue fraction, without whole muscle or individual fiber atrophy, has been demonstrated in the multifidus in an experimental model of intervertebral disc injury.49 These studies highlight the important role that tissue composition may play in the clinical manifestation of spinal musculoskeletal pathology. Although decreased muscle quality has been observed in individuals with low back pain and injury, there is also an age-associated increase in fatty infiltration that appears to be independent of pathology. Recent data demonstrates that age accounts for approximately 30% of the variance in fatty infiltration in the multifidus and erector spinae muscles (Fig. 9-5).50 The causative relationships (if any) among age, tissue composition, spinal musculoskeletal pathology, and clinical manifestation of pain and dysfunction remains an open question.
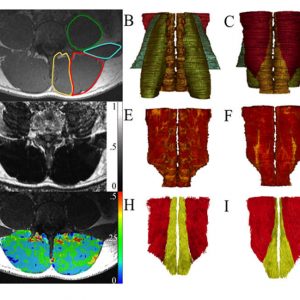
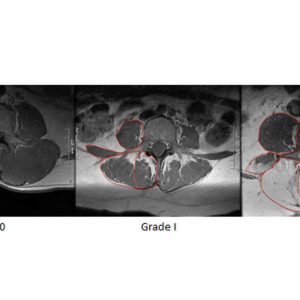
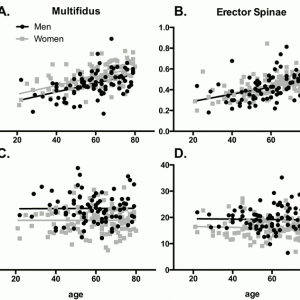
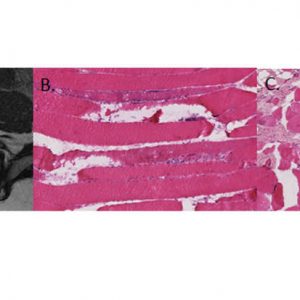
The biological processes underlying gross fatty infiltration have yet to be elucidated. However, existing literature suggests that one of the primary modes of decline in muscle function is atrophy, which is defined as the loss of contractile protein volume (reduced fiber area) due to reduced loading demands on the muscle. Evidence of lumbar muscle atrophy has been reported at the whole muscle and individual fiber level in the form of decreased CSA,51,52 although evidence for simple muscle atrophy is conflicting.49 Muscle atrophy may also be fiber type specific, as selective atrophy of type 2 fibers was reported along with an increase in the proportion of transitional fibers.51,53,54 These atrophic changes could result from denervation, pain, or disuse-related inactivation of the lumbar musculature. Experimental evidence suggests that the lumbar multifidus undergoes rapid atrophy following disc and spinal nerve root injury with distinct patterns of atrophy associated with the specific etiology.55 Disc injury resulted in single level atrophy of the multifidus and was hypothesized to be secondary to reflex inhibitory mechanisms and subsequent disuse. Nerve root injury resulted in multi-level atrophy of the multifidus, consistent with the known innervation pattern of the multifidus muscle.
Importantly, muscle degeneration, the process of active necrosis and cell death due to cell membrane disruption, may also contribute to contractile volume loss in individuals with lumbar spine pathology. This degeneration may be a consequence of chronic unloading-related atrophy, or it may be in addition to atrophy. Either way, this represents a quantitative loss of contractile tissue in addition to, or reflective of, qualitative changes within the contractile tissue. It also may explain why, in some cases, exercise-related rehabilitation is not successful in reducing fatty infiltration. Both atrophy and degeneration can be observed histologically from biopsies of the lumbar musculature (Fig. 9-6). The mechanisms responsible for atrophy versus degeneration are still unknown, although it is commonly thought that both ultimately result in the irreversible replacement of functional contractile tissue with fat.
SUMMARY
Muscle function in the lumbar spine is influenced by muscle anatomy, architecture, and quality. Muscular architecture is an important, and often overlooked, determinant of muscle function because it interacts with the skeletal and nervous systems in complex ways. Architectural and biological factors determining muscle quality must be examined together to fully understand the biomechanical function of a muscle and its contribution to any pain or injury mechanisms. Detailed anatomic and architectural studies have yielded insights into spinal muscle functions, but the architecture of many spinal muscles still needs to be examined. Furthermore, the mechanisms contributing to muscle quality changes associated with lumbar spine pathology are also incompletely understood. All of these data are necessary for accurate biomechanical models, which must be used in conjunction with experimental studies to elucidate the function of spinal muscles and their role in pathologic processes of the spine. We believe that this information can ultimately be used to provide accurate diagnoses and prognoses, to optimize surgical decision making, and to develop improved prevention and rehabilitation strategies that optimize function.
REFERENCES
- Panjabi MM. The stabilizing system of the spine. Part I. Function, dysfunction, adaptation, and enhancement. J Spinal Disord. 1992;5(4):383-389; discussion 397.
- Gans C. Fiber architecture and muscle function. Exerc Sport Sci Rev. 1982;10:160-207.
- Lieber RL, Friden J. Functional and clinical significance of skeletal muscle architecture. Muscle Nerve. 2000;23(11):1647-1666.
- Gans C, Bock WJ. The functional significance of muscle architecture--a theoretical analysis. Ergeb Anat Entwicklungsgesch. 1965;38:115-142.
- Gans C, de Vree F. Functional bases of fiber length and angulation in muscle. J Morphol. 1987;192(1):63-85.
- Lieber RL. Skeletal Muscle Structure and Function: Implications for Physical Therapy and Sports Medicine. Baltimore: Williams & Wilkins;1992.
- Bodine SC, Roy RR, Meadows DA, et al. Architectural, histochemical, and contractile characteristics of a unique biarticular muscle: the cat semitendinosus. J Neurophysiol. 1982;48(1):192-201.
- Ounjian M, Roy RR, Eldred E, et al. Physiological and developmental implications of motor unit anatomy. J Neurobiol. 1991;22(5):547-559.
- Loeb GE, Pratt CA, Chanaud CM, Richmond FJ. Distribution and innervation of short, interdigitated muscle fibers in parallel-fibered muscles of the cat hindlimb. J Morphol. 1987;191(1):1-15.
- Lieber RL, Loren GJ, Friden J. In vivo measurement of human wrist extensor muscle sarcomere length changes. J Neurophysiol. 1994;71(3):874-881.
- Llewellyn ME, Barretto RP, Delp SL, Schnitzer MJ. Minimally invasive high-speed imaging of sarcomere contractile dynamics in mice and humans. 2008;454(7205):784-788.
- Cromie MJ, Sanchez GN, Schnitzer MJ, Delp SL. Sarcomere lengths in human extensor carpi radialis brevis measured by microendoscopy. Muscle Nerve. 2013;48(2):286-292.
- Burkholder TJ, Lieber RL. Sarcomere length operating range of vertebrate muscles during movement. J Exp Biol. 2001;204(Pt 9):1529-1536.
- Lieber RL, Ljung BO, Friden J. Intraoperative sarcomere length measurements reveal differential design of human wrist extensor muscles. J Exp Biol. 1997;200(Pt 1):19-25.
- Rome LC, Sosnicki A, Choi IH. The influence of temperature on muscle function in the fast swimming scup. II. The mechanics of red muscle. J Exp Biol. 1992;163:281-295.
- Rome LC, Sosnicki AA. Myofilament overlap in swimming carp. II. Sarcomere length changes during swimming. Am J Physiol. 1991;260(2 Pt 1):C289-296.
- Kalimo H, Rantanen J, Viljanen T, Einola S. Lumbar muscles: structure and function. Ann Med. 1989;21(5):353-359.
- Macintosh JE, Bogduk N. 1987 Volvo award in basic science. The morphology of the lumbar erector spinae. Spine (Phila Pa 1976). 1987;12(7):658-668.
- Delp SL, Suryanarayanan S, Murray WM, Uhlir J, Triolo RJ. Architecture of the rectus abdominis, quadratus lumborum, and erector spinae. J Biomech. 2001;34(3):371-375.
- Ward SR, Kim CW, Eng CM, et al. Architectural analysis and intraoperative measurements demonstrate the unique design of the multifidus muscle for lumbar spine stability. J Bone Joint Surg Am. 2009;91(1):176-185.
- Regev GJ, Kim CW, Thacker BE, et al. Regional Myosin heavy chain distribution in selected paraspinal muscles. Spine (Phila Pa 1976). 2010;35(13):1265-1270.
- Polgar J, Johnson MA, Weightman D, Appleton D. Data on fibre size in thirty-six human muscles. An autopsy study. J Neurol Sci. 1973;19(3):307-318.
- Macintosh JE, Valencia F, Bogduk N, Munro RR. The morphology of the human lumbar multifidus. Clin Biomech (Bristol, Avon). 1986;1(4):196-204.
- Macintosh JE, Bogduk N. The biomechanics of the lumbar multifidus. Clin Biomech (Bristol, Avon). 1986;1(4):205-213.
- Thorstensson A, Carlson H. Fibre types in human lumbar back muscles. Acta Physiol Scand. 1987;131(2):195-202.
- McGill S, Juker D, Kropf P. Quantitative intramuscular myoelectric activity of quadratus lumborum during a wide variety of tasks. Clin Biomech (Bristol, Avon). 1996;11(3):170-172.
- McGill SM, Patt N, Norman RW. Measurement of the trunk musculature of active males using CT scan radiography: implications for force and moment generating capacity about the L4/L5 joint. J Biomech. 1988;21(4):329-341.
- Santaguida PL, McGill SM. The psoas major muscle: a three-dimensional geometric study. J Biomech. 1995;28(3):339-345.
- Juker D, McGill S, Kropf P, Steffen T. Quantitative intramuscular myoelectric activity of lumbar portions of psoas and the abdominal wall during a wide variety of tasks. Med Sci Sports Exerc. 1998;30(2):301-310.
- Bogduk N, Johnson G, Spalding D. The morphology and biomechanics of latissimus dorsi. Clin Biomech (Bristol, Avon). 1998;13(6):377-385.
- McGill SM, Hughson RL, Parks K. Changes in lumbar lordosis modify the role of the extensor muscles. Clin Biomech (Bristol, Avon). 2000;15(10):777-780.
- Norman R, Wells R, Neumann P, Frank J, Shannon H, Kerr M. A comparison of peak vs cumulative physical work exposure risk factors for the reporting of low back pain in the automotive industry. Clin Biomech (Bristol, Avon). 1998;13(8):561-573.
- Lieber RL, Thornell LE, Fridén J. Muscle cytoskeletal disruption occurs within the first 15 min of cyclic eccentric contraction. J Appl Physiol (1985). 1996;80(1):278-284.
- Lieber RL, Fridén J. Mechanisms of muscle injury after eccentric contraction. J Sci Med Sport. 1999;2(3):253-265.
- McGill SM, Norman RW. Effects of an anatomically detailed erector spinae model on L4/L5 disc compression and shear. J Biomech. 1987;20(6):591-600.
- Quint U, Wilke HJ, Shirazi-Adl A, Parnianpour M, Löer F, Claes LE. Importance of the intersegmental trunk muscles for the stability of the lumbar spine. A biomechanical study in vitro. Spine (Phila Pa 1976). 1998;23(18):1937-1945.
- Crisco JJ 3rd, Panjabi MM. The intersegmental and multisegmental muscles of the lumbar spine. A biomechanical model comparing lateral stabilizing potential. Spine (Phila Pa 1976). 1991;16(7):793-799.
- Cholewicki J, McGill SM. Mechanical stability of the in vivo lumbar spine: implications for injury and chronic low back pain. Clin Biomech (Bristol, Avon). 1996;11(1):1-15.
- Hamberg-van Reenen HH, Ariens GA, Blatter BM, Twisk JW, van Mechelen W, Bongers PM. Physical capacity in relation to low back, neck, or shoulder pain in a working population. Occup Environ Med. 2006;63(6):371-377.
- Zabihhosseinian M, Holmes MW, Ferguson B, Murphy B. Neck muscle fatigue alters the cervical flexion relaxation ratio in sub-clinical neck pain patients. Clin Biomech (Bristol, Avon). 2015;30(5):397-404.
- Nimbarte AD, Zreiqat MM, Chowdhury SK. Cervical flexion-relaxation response to neck muscle fatigue in males and females. J Electromyogr Kinesiol. 2014;24(6):965-971.
- Uhlig Y, Weber BR, Grob D, Muntener M. Fiber composition and fiber transformations in neck muscles of patients with dysfunction of the cervical spine. J Orthop Res. 1995;13(2):240-249.
- Mazis N, Papachristou DJ, Zouboulis P, Tyllianakis M, Scopa CD, Megas P. The effect of different physical activity levels on muscle fiber size and type distribution of lumbar multifidus. A biopsy study on low back pain patient groups and healthy control subjects. Eur J Phys Rehabil Med. 2009;45(4):459-467.
- Brown SH, Gregory DE, Carr JA, Ward SR, Masuda K, Lieber RL. ISSLS prize winner: adaptations to the multifidus muscle in response to experimentally induced intervertebral disc degeneration. Spine (Phila Pa 1976). 2011;36(21):1728-1736.
- Crossman K, Mahon M, Watson PJ, Oldham JA, Cooper RG. Chronic low back pain-associated paraspinal muscle dysfunction is not the result of a constitutionally determined "adverse" fiber-type composition. Spine (Phila Pa 1976). 2004;29(6):628-634.
- Teichtahl AJ, Urquhart DM, Wang Y, et al. Fat infiltration of paraspinal muscles is associated with low back pain, disability and structural abnormalities in community-based adults. Spine J. 2015;15(7):1593-1601.
- Käser L, Mannion AF, Rhyner A, Weber E, Dvorak J, Müntener M. Active therapy for chronic low back pain: part 2. Effects on paraspinal muscle cross-sectional area, fiber type size, and distribution. Spine (Phila Pa 1976). 2001;26(8):909-919.
- Airaksinen O, Herno A, Kaukanen E, Saari T, Sihvonen T, Suomalainen O. Density of lumbar muscles 4 years after decompressive spinal surgery. Eur Spine J. 1996;5(3):193-197.
- Hodges PW, James G, Blomster L, et al. Multifidus muscle changes after back injury are characterized by structural remodeling of muscle, adipose and connective tissue, but not muscle atrophy: Molecular and morphological evidence. Spine (Phila Pa 1976). 2015;10(14):1057-1071.
- Shahidi B, Parra CL, Berry DB, et al. Contribution of lumbar spine pathology and age to paraspinal muscle size and fatty infiltration. Spine (Phila Pa 1976). 2017;42(8):616-623.
- Mannion AF, Dumas GA, Cooper RG, Espinosa FJ, Faris MW, Stevenson JM. Muscle fibre size and type distribution in thoracic and lumbar regions of erector spinae in healthy subjects without low back pain: normal values and sex differences. J Anat. 1997;190 (Pt 4):505-513
- Ng JK, Richardson CA, Kippers V, Parnianpour M. Relationship between muscle fiber composition and functional capacity of back muscles in healthy subjects and patients with back pain. J Orthop Sports Phys Ther. 1998;27(6):389-402.
- Mannion AF, Weber BR, Dvorak J, Grob D, Müntener M. Fibre type characteristics of the lumbar paraspinal muscles in normal healthy subjects and in patients with low back pain. J Orthop Res. 1997;15(6):881-887.
- Mannion AF. Fibre type characteristics and function of the human paraspinal muscles: normal values and changes in association with low back pain. J Electromyogr Kinesiol. 1999;9(6):363-377.
- Hodges P, Holm AK, Hansson T, Holm S. Rapid atrophy of the lumbar multifidus follows experimental disc or nerve root injury. Spine (Phila Pa 1976). 2006;31(25):2926-2933