Daniel Haschtmann and Stefan Dudli
INTRODUCTION
Until now, the question whether spine trauma in the specific case is associated with damage of the intervertebral disc that ultimately leads to organ degeneration, remains controversial. There are conflicting data in clinical as well as in experimental studies.
The biological cues of posttraumatic disc degeneration (DD) and idiopathic disc degeneration are different. While posttraumatic DD is triggered by a single traumatic event, idiopathic DD is the result of accumulated damage, which relates to mechanical, genetic and environmental risk factors.1,2 Clinical trauma studies face the problem that the degenerative process, which is initiated by a traumatic event can take a long time to evolve which then may or may not become symptomatic with a new onset of pain and/or neurological symptoms. MR imaging in these cases may eventually show degenerated intervertebral discs. If these discs are adjacent to formerly fractured vertebra and the other discs are healthy, a traumatic aetiology may be assumed. However, if the fractures are healed without a deformity or only residual minor endplate changes such as Schmorl’s nodes, it remains difficult to separate these from a developmental or idiopathic degenerative genesis. It becomes even more difficult to evaluate the effects of traumatic events to the spine (and discs) without a bony fracture, instantly visible damage of the disc organ or idiopathic disc degeneration that was already present.
In spine trauma, any direction of force induction into the intervertebral disc such as compression, distraction and rotation/translation can lead to a disc injury or disc failure depending on the involved energy. The intervertebral disc can be affected primarily by damaging or loss of organ tissue or, secondarily, through metabolic, immunological or biomechanical changes, such as segmental instability with subsequent damage the disc organ. The damage can range from a non-detectable injury over limited traumatic annular tears or nucleus pulposus prolapse to a complete destruction of the disc organ such as in fracture dislocations. Depending on the involved tissues, i.e. annulus fibrosus, nucleus pulposus and vertebral endplates, different trauma type-dependent alterations in mechanical load distributions and co-location of the immune-privileged nucleus pulposus (NP) with nerve roots or the bone marrow are created. Both, mechanical load shifts and exposure of the NP to immune surveillance initiate biological reactions that are injury type specific.
The currently used subaxial and thoracolumbar classifications for spine injuries are based on the injury mechanisms, resulting morphological and biomechanical changes of the motion segment and the presence of neurological injury.3 However, there is currently no classification available that covers the entire spectrum of injuries focused on the intervertebral disc. Therefore, a disc injury classification with three different basic injury types was established considering the grade of destruction of the different disc components based on morphology and injury mechanism (Table 7-1/Fig. 7-1). In the following paragraphs the injury types are described in detail and where available validated with clinical and biological experimental studies. It must be noted that in an individual case a clear distinction between the injury types may not always be possible and there may be overlapping. In a specific clinical case, it is often difficult to fully assess disc damage as information on the direction of involved forces and the amount of induced energy are incomplete (Fig. 7-2 A-C). Furthermore, biomechanics differ significantly in the specific regions of the spine and the different imaging modalities, biomechanical testing measures and histological as well as immunological methods are evolving.
A. | Non-destructive intervertebral disc injury without loss of NP tissue
|
B. | Partially-destructive intervertebral disc injury with or without loss of NP tissue
|
C. | Destructive intervertebral disc injury (organ disruption)
|
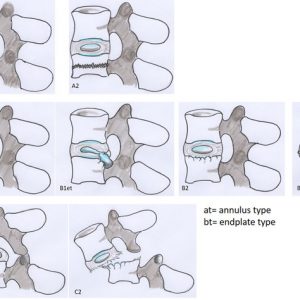
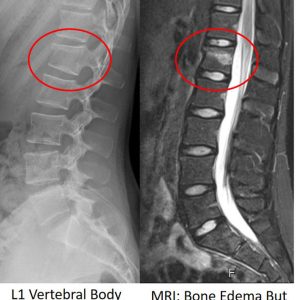
17 year old girl after fall from horseback. Imaging shows a L1 vertebral body fracture with a minor kyphotic deformity. Magnetic resonance imaging (MRI) demonstrates an oedema within the vertebra but the endplate remained intact. The disc injury is classified as type A2.
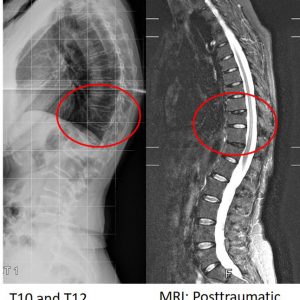
66 year old woman with two healed osteoporotic compression fractures at T10 and T12. Imaging shows posttraumatic disc degeneration at T10/T11 and T12/L1 after disc injury type B2. Note that the lumbar spine does not show any evidence of idiopathic disc degeneration.
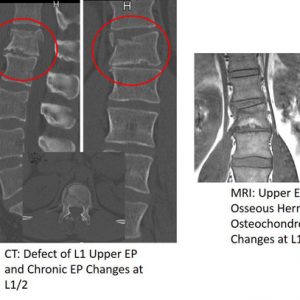
36 year old man after ski accident. Imaging including computed tomography (CT) shows an upper endplate burst fracture of L1 and a disc injury type B3 at T12/L1. Note the defect and fracture lines in the L1 upper endplate and the morphological similarities to the preexisting osteochondrosis at the segment below (L1/2).
For the clinical assessment of posttraumatic disc degeneration there are currently two different main types of studies available employing MRI as surrogate for disc health.4 First, observational studies that demonstrate the natural history after spine trauma of patients treated conservatively with the MRI performed in the later course. Second, patients undergoing surgery with posterior instrumentation but retention of the disc and the MRI done with either the spinal fixator in place or after implant removal. In the latter group, it is of importance if posterior fusion was applied as dorsal bony bridging most likely alters the loading of the disc. For example, extended posttraumatic structural disc disruption with endplate defects and huge herniation of nucleus material through the defect would in most cases result in disc degeneration. However, if the segment is stabilized with posterior instrumentation and thus the disc is stress-shielded, the compartment may be allowed to heal. Similar to a stabilization of a segment with idiopathic disc degeneration using a dynamic posterior implant where a recovery of the nucleus signal can be observed in some cases,5 it has also been shown that, after posteriorly stabilized burst fractures, the nucleus signal may be retained even after implant removal.6 This highlights the potentially detrimental role of posttraumatic loading. In all cases, it is crucial when the MRI was performed, not only because degenerative changes may take time to evolve, but also because acute traumatic disc alteration, such as bleeding into the disc compartment or disc oedema, may mask other structural or degenerative changes.7
Acute disc damage must be separated from disc function, from repair mechanisms and from posttraumatic as well as overlapping idiopathic degeneration.6 Sander et al. have established a MRI classification for disc injuries which can be used for acute as well as chronic posttraumatic changes (Fig. 7-3/ Table 7-2).8
Grade | MRI T1 weighted | MRI T2 weighted/T2 TIRM | Characteristic morphologic finding | |
0 | Intact | |||
1 | Hyperintense | Edema | ||
2 | Isointense to hyperintense | Hypointense with perifocal hyperintense appearence | Disc rupture with intradiscal bleeding | |
3 | Isointense to hyperintense | Hypointense with perifocal hyperintense appearence | Infraction of the disc into the vertebral body, annular tears or infraction without herniation into endplate |
Thus far, it has not proven that disc repair is possible at all in the adult population. If repair is provided it may be linked with the (genetic) disposition for idiopathic disc degeneration. On the other hand, if disc repair is not provided, it may be accepted that, even in a burst fracture with creep of the disc into the underlying vertebra, disc damage may only be minor, and the disc can continue to at least partially function.
Taken together, significant spine trauma creates an altered mechanical, cellular and metabolic status and may lead to cell death and malfunction of the disc compartments. Subsequently, repair or degeneration occur, which depend on multiple factors such as the type and extent of damage, the immune system, the genetic susceptibility to disc degeneration, which is linked with age, the biomechanical (functional) condition that is created by the injury, and finally the type of treatment: conservative (disc loading) or operative (disc unloading).
In-Vitro and In-Vivo Models for Posttraumatic Disc Degeneration
There is currently no genuine in-vivo model for posttraumatic disc degeneration (PTDD) because of ethical considerations and questionable reproducibility. However, PTDD without impact loading can be induced effectively in animal models by means of endplate perforation or by annulus fibrosus (AF) laceration.9-11 However, these are artificial trauma types and lack the high-energy impact that associate with human disc failure. Explant of human or animal spinal segments have proven extremely valuable, because they overcome the ethical limitation of applying high energy trauma in-vivo and because the culture environment is largely controllable without losing the native tissue and organ structure.12 Trauma can be reproducibly induced and posttraumatic conditions are controllable.13 Furthermore, explants are cost effective, because a maximal use of tissue is achieved. Retaining the endplate has been shown to be pivotal to restrict tissue swelling and osmotic cell death.14,15 Disc/endplate specimens from small animals can be cultured without losing cell phenotype for up to 4 weeks.16 Cyclic loading during culture period is generally superior to no or static loading, probably because it enhances fluid flow and cell nutrition, but also because it directly exerts a mechanobiological stimulus on cells.17 Almost all discs from laboratory animals are smaller than human discs; they have a higher cellularity and, hence, have a higher healing capacity and a lower risk to accumulate disc damage, which results in disc degeneration.16 Furthermore, notochordal cells in rodents and pigs also support healing mechanisms. In summary, in-vivo and explant models of animals are well capable to study posttraumatic disc degeneration after major trauma or repeated trauma but are not well suited to study the long-term consequences after minor trauma due to different spinal loads and different healing capacities.
Non-Destructive Intervertebral Disc Injury without Loss of Nucleus Pulposus Tissue, Disc Injury Type A
In injury type A1, a minor intervertebral disc injury without loss of nucleus pulposus is usually caused by low energy trauma. The disc is loaded with compression (contusion) or shear/distraction, but the annulus remains intact and functional; the nucleus is contained. There are no fractures or other morphological changes of the disc organ, the endplates or vertebral body detectable with the current imaging techniques available. In type A2 disc injury, a fracture within the vertebral body or the opposite endplate (AO type A fracture) but away from the intervertebral disc of interest has occurred.
Clinical studies injury type A
Although theoretically possible, there are not currently any clinical studies showing concurrent disc degeneration after low energy spine trauma without any detectable damage of the vertebral bone including the endplates or intervertebral disc. In a retrospective study, thirty-eight exposure-discordant monocygotic twins who sustained painful back injury in the past related to work, leisure or sport were interviewed. The authors did not find any difference in disc degeneration using MRI at a mean follow up of 18.6 years. As a limitation, the series was relatively small and no information on the type of trauma and the involved energy as well as trauma imaging was available. Furthermore, cases with major trauma including fractures were excluded.18 The results are in accordance with Sander et al. who found that intervertebral discs away from the fracture sides did not develop any MRI signal changes after 1 year.7 A criticism may be that the follow-up interval may have been too short for a definitive estimation.
Shear and distraction injuries of the intervertebral disc are well established in the literature, especially for the cervical spine. Depending on the involved loads and direction of force, it can range from a subclinical strain of some annular fibers (whiplash injury) that would be referred to the disc injury type A1 over a traumatic disc herniation (injury type B) to a complete dislocation with separation of the segment, disruption of the annular fibers, longitudinal ligaments, joint capsule and posterior structures (disco-ligamentous complex, DLC) that would be injury type C.19
Despite some evidence from biomechanical cadaver studies,20 currently no association between a whiplash injury and posttraumatic disc degeneration is established for the cervical spine in vivo.21 The methodical difficulty in this context consists of the large prevalence of disc degeneration in the asymptomatic population.22
A controlled study with an 11 -year follow up investigated the development of Modic changes as a marker for disc degeneration after whiplash injury using MRI. In comparison with asymptomatic volunteers, no association between Modic changes and trauma was found but rather with pre-existing disc degeneration.23
In the situation of a vertebral fracture (injury type A2) but intact disc/endplate complex and in the presence of sufficient bone quality, the involved trauma energy can by assumed to be much higher than in the aforementioned twin study from Hancock and Battié. Here the clinical investigations are contradictory. In a clinical study by Kerttula et al. with a follow-up of 4.3 years, no increased rate of disc degeneration was found on MRI if the vertebral endplates were intact.24 It must be mentioned that the study had a low patient number of n = 14 and was performed in children with a mean age of 11.8 years when trauma occurred. In contrast, in another MRI study in adult spine fracture patients, it was demonstrated that the caudal intervertebral discs adjacent to the fractured vertebra but with an intact endplate, almost half of the discs demonstrated instant MR signal changes. Moreover, if in this study MRI signal intensity was found initially to be normal (grade 0 lesion) in approx. half of the patients the discs demonstrated degeneration to grade 2 lesions after 1 year.7 Here, it may be criticized that the follow-up interval for MRI assessment may have been too short for a definitive estimation.
Experimental studies injury type A
In an experimental in-vivo rattail model, intervertebral discs were loaded for 1.5 hours with approximately 300% body weight (12.6 N) at 1 Hz. The mechanosensitive disc cells responded with an up-regulation of different anabolic but also catabolic genes such as aggrecan, TIMP-1, ADAMTS-4, MMP-3 and MMP-13.25 This gene pattern implies that high loading can already be regarded as a traumatic event to the disc resulting in tissue turnover, remodeling and repair, or even injury. In another study using rabbit disc explants, a traumatic disc event without structural damage to the NP, AF and endplate caused transcriptional up-regulation of pro-inflammatory cytokines and catabolic enzymes.26 In this model the changes normalized within a few days and no morphological alterations were found up to 4 weeks. However, high dynamic compressive loads may also cause micro-cracks to the endplates, microscopically delaminate and tear the AF. These damages may propagate over time and predispose the structure to failure under continuous loading.27
Vertebral body fracture without the involvement of the endplate (type A2), the NP or the AF does not directly affect the disc structure, and, hence, the direct biological consequences may be assumed to be similar to injury type A1. However, biomechanical studies have shown that the induced force to create vertebral body fractures away from the endplates, depending on the segmental flexion angle, can be even higher than for the creation of an endplate fracture.28 Moreover, a biomechanical study using discomanometry has shown that not only the discs adjacent to complete vertebral burst fractures but also the non-adjacent discs at the next lower segments were injured.29 Therefore, also a higher rate of cell death and inflammatory changes may be expected. Unfortunately, there is currently no animal model or explant model to study biological changes and if repair can take place in discs after type A2 trauma.
Biomechanical consideration also supports the notion of posttraumatic DD after type A2 trauma. Vertebral body fractures decrease vertebral strength30 by reducing trabecular interconnectivity.31-33 The loss of the load-carrying capacity of the vertebra can cause non-recoverable endplate deflections and endplate damage under compressive loads, which precede DD.34 In summary, unlike in clinical studies, and, although transient biological changes can be detected, disc trauma without loss of nucleus tissue or nucleus herniation and without endplate impairment so far has not shown to promote disc degeneration in biological models. However, non-detected endplate fissures and bony changes away from the disc may make the disc susceptible to degeneration under repeated dynamic loads.
Partially-Destructive Intervertebral Disc Injury with or without Loss of Nucleus Pulposus Tissue, Disc Injury Type B
In injury type B, an intervertebral disc injury is caused either by a focal rupture of the annulus fibrosus, failure of the annulus endplate junction or a fracture of the vertebral endplate. The disc is loaded with compression or shear/distraction, and nucleus pulposus tissue is lost as it herniates either through the ruptured annulus or failed annulus-endplate interface (disc injury type B1) or through the fractures lines of the endplate (disc injury type B3). Depending on the extent of endplate fracture and NP herniation the damage ranges from minor when only small fracture lines are evident to a virtual loss of the entire nucleus tissue to the vertebral bone. Even if NP is not fully contained, the disc organ may remain at least partially functional.
An endplate fracture can impair the intervertebral disc in several ways. Biomechanical studies using human motion segments have been shown that endplate fracture can already occur with an axial force (0.5 mm/sec) of 4.5 kN depending on the spinal alignment.35 In a burst fracture the intravertebral pressure increases and the bony fragments are pushed centrifugally apart, which weakens the anchorage of the annulus as well as creates gaps between the fragments to allow the nucleus to herniate into the underlying bone.28,29 If the fragments are additionally depressed, the disc containment is impaired and even nucleus material can be lost into the underlying trabecular bone. In contrast, a simple fracture line in the endplate that is closed maybe less problematic and no or only minor loss of NP material is expected. However, if the split goes through the whole vertebral body the resulting instability not only stresses the annular attachment, but also a substantial loss of NP tissue may be possible. In these AO type A2.2 cases, Sander et al. have observed a 100% rate of disc degeneration.7 The consequence of an endplate fracture is a possible defect healing which abrogates the function of the endplate for disc nutrition and thus promotes disc degeneration. The velocity and severity of the degenerative process most likely depends on the function of the opposite endplate.
Clinical studies injury type B1
Traumatic disc herniation is frequently encountered in the cervical spine and can occur isolated (type B1) or together with fractures or facet dislocations (type C).36 In the former case, the nucleus pulposus herniates through the annular fibers that were torn by distraction or weakened by pre-existing degeneration.37 If not treated surgically, a degenerative process can be observed.38 Traumatic NP herniation can occur in the setting of pre-existing DDD or in a previous healthy disc. It can present as an isolated annulus rupture or combine with a fracture, facet dislocation or failure of the annular anchorage. Organ damage is often substantial, and a restitution ad integrum is unlikely. While there is not much doubt that traumatic disc herniation can occur in a degenerated segment,5 and, although documented in biomechanical in-vitro studies,39 the existence of an isolated annulus fiber rupture with NP herniation caused by a single traumatic event without (proven) pre-existing DD is still debated in the clinical literature.
Any kind of annular defect is linked with disc degeneration that is either pre-existing or initiated by trauma. This evidence is supported by a clinical investigation from Carragee et al. demonstrating that an annular needle puncture and disc injection, e.g., discography, results in an accelerated disc degeneration in normal as well as moderately degenerated discs compared to a matched control. Using MRI, a reduction of NP signal intensity and disc height as swell as a higher rate of disc herniation could be documented in 35% of the injected discs compared to 14% in the control group.40 In a clinical study by Rajasekaran et al., the anatomy of adult lumbar disc herniation was investigated to document the site of disc failure using different image modalities and clinical, as well histological, examinations of surgically retrieved disc specimens. In a series of 181 patients with lumbar disc herniations in 65% of the cases the site of failure was the annulus endplate junction with bony changes or avulsions at the posterior rims of the endplates.41 This is similar to traumatic disc herniation with avulsion of the ring apophysis in the growing skeleton while ossification of the growth plates occur.42,43 According to Takata et al., these physis fractures can be classified depending on the size of the fragment and involvement of the vertebral body.44 In a biomechanical study using young porcine functional spine units endplate/apophysis fractures were induced with intradiscal pressurization. The specimens additionally demonstrated decreased laminate adhesion between the posterior AF layers indicating annular damage.45 Obvious similarities exist between the described traumatic avulsion of the ring apophysis in adolescents and the annulus endplate junction failure in adults (Fig. 7-4) as described by Rajasekaran.41
Experimental Studies Injury Type B1
Experimental studies have shown that herniation of the nucleus pulposus (NP) through the defect annulus fibrosus (AF) triggers a series of biomechanical and biological responses. The depressurization of the NP causes a load shift from the NP to the AF under compressive loads.46 Consequently, the inner AF fibers collapse into the depressurized NP space. Redistribution of compressive stresses causes regions of low and high compressive stresses, both of which are known to inhibit disc cell metabolism47 and thereby increase the risk of internal disruption and degeneration.48 The ability of the few remaining disc cells to repair the damage of a vast matrix is further reduced by altered matrix stresses. The result is a vicious cycle of frustrated healing attempts and repeated re-injury.49 The discs attempt to heal the damage causes a switch from collagen type II and aggrecan towards collagen type I, versican, biglycan and decorin.50 Annular stabbing with needles in animal model consistently triggers disc degeneration, characterized by loss of disc height, hydration and proteoglycans and by the expression of inflammatory, catabolic and fibrotic proteins.16 Although, the NP does not herniate in these models and, hence, does not cause a shift in load distribution, the consequences of a single traumatic event with structural damage to the AF and NP can be investigated. Therefore, annular stabbing are valid models to investigate posttraumatic disc herniation.16,51,52
Herniated discs trigger an innate and adaptive immune response with infiltration of macrophages, T and B cells, and the up-regulation of a myriad of pro-inflammatory cytokines, e.g., IL-1, IL-6, IL-8, IL-10, IL-17, IFN-γ, CCL2, TNF-α and PGE2.53,54 Importantly, IL-6, IL-8, and PGE2 correlate with pre-operative sciatic symptoms.54 Sciatic pain also relates to mechanical displacement of the nerve root and to a lesser extent to the displacement of the dorsal root ganglion as shown in a rat model.55 Animal models to study the immunological consequences after disc herniations require surgical displacement of NP into immune surveilled tissue.56
Microbial infection of herniated discs, in particular with Gram-positive bacteria such as Cutibacterium acnes (C. acnes), can cause inflammation around the nerve roots.57 The herniation trajectory may also provide a way for the bacteria to infiltrate the disc, aggravate degeneration and cause Modic changes.58,59 C. acnes not only activate immune cells but also trigger a pro-inflammatory response of disc cells.60 Furthermore, the C. acnes virulence factors lipase, hyaluronidase and neuraminidase are pro-inflammatory and may add to disc degenerative changes.
Clinical studies injury type B2 and B3
In the cervical as well as the thoracolumbar spine in the case of an endplate fracture, nucleus material is lost through the fracture lines or depressed fragments of the endplate either instantly or with continuous loading. It must be distinguished between a limited EP damage with relatively stable fragments, closed fracture lines or small defects, which result in no or a limited loss of nucleus tissue (injury type B2), from large defect with a virtual complete loss of NP tissue into the vertebral bone (type B3). In the first case, the disc remains partially functional, but subsequent degeneration may occur. This has been shown in a trauma study with 14 children by Kerttula et al. who demonstrated degenerative disc changes after mean 3.4 years in 8 patients, all being older than 15 years. In seven patients, disc degeneration occurred at the injured vertebra, and, in six cases, irregularities of the vertebral endplates were evident. In the same study, all children below 15 years of age with vertebral fractures did not show any degenerative disc changes on MRI.24 This is partially in accordance with a study by Moller et al. who assessed the risk for DD 40 years after spine fractures in childhood (4-16 years age) using MRI. The authors also did not find any increase of degeneration of intervertebral discs adjacent to the fractures compared to the controls in this young cohort. However, endplate defects, e.g., Schmorl’s nodes, were noted more frequently.61 In another MRI study by Markku Vornanen et al., it was shown that in well selected population (n = 14) of non-pediatric patients (from n = 122) with thoracolumbar burst fractures or flexion distraction injuries, in 11 patients degeneration with signal loss on MRI, one or both discs adjacent to a fractured vertebra occurred, and, in all concerned discs, a decreased height was evident after long segment instrumentation and later implant removal. However, in three patients, the signal intensities of all discs were normal but disc height was reduced at least in one of the two adjacent discs. Of importance, all discs away from the fracture were normal. Unfortunately, in this study, no analysis with respect to fracture type and involvement of both endplates and extent of endplate damage was done.62
Based on a study by Battié et al., Oner et al. developed a classification of disc changes from 38 thoracolumbar fractures in 35 patients encompassing 6 types based on morphological and MRI changes63 (Fig. 7-5A, B). The authors classified 75 fractures in 63 patients treated either conservatively (n = 26) or with posterior instrumented fusion and later implant removal. They found that in approx. 80% the discs caudal from the fracture were normal or had Schmorl’s nodes with preserved nucleus signal. The cranial discs exhibited a signal loss of the NP in approx. 25% and an osseous nucleus herniation through the endplate in approx. 36% but retained nucleus signal. Unfortunately, the classification does not allow an accurate separation between morphological changes (e.g. endplate defects) and MRI signal changes. Furthermore, in the surgically treated group a posterior fusion was carried out which has shown to unload the disc (Fig. 7-5A, B).
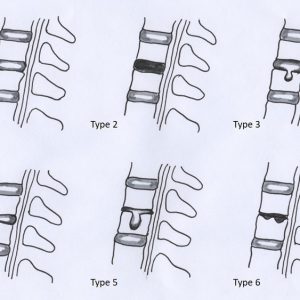
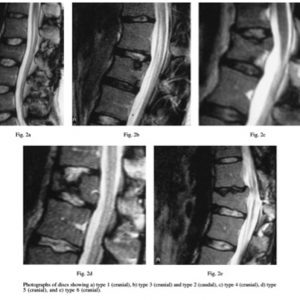
In a study by Hsu and Zucherman et al., high lumbar disc degeneration was analyzed using MR imaging. The authors found that isolated disc degeneration in the upper lumbar spine was associated with pre-existing endplate defects and vertebral fractures adjacent to the respective intervertebral discs.64 This is in accordance with prospective MRI study by Fürderer et al. on spine trauma patients undergoing posterior spinal fracture stabilization and later implant removal. They found instant posttraumatic MR signal changes in 60% of the intervertebral discs. Moreover, in 37.5% of the discs, a generation occurred as assessed after implant removal.6
Experimental Studies Injury Type B2 and B3
Osseous NP herniation can occur after endplate fracture or Schmorl’s nodes; both of which are associated with disc degeneration. 24,65 However, Schmorl’s nodes may have different aetiologies, and cause-response in relation to disc degeneration remains a question of debate.66 Endplate fracture and Schmorl’s node defects cause the expulsion of a part of the NP into the marrow space. This causes depressurization of the NP and inward collapse of AF fibers, which causes degeneration similar to annular disc herniation. Loss of disc height, hydration and proteoglycans has also been reported after experimental endplate perforation in pigs and endplate fracture in rabbit spinal explants.13,67 In this model, fracture of the endplate causes an immediate and broad up-regulation of pro-inflammatory cytokines in the NP and to a lesser extent in the AF. The up-regulation of TNF-α, interleukin (IL)-1, IL-5, IL-6, IL-7, and the chemokines IL-8, monocyte chemotactic protein (MCP)-1, MCP-2, GROα, MIG26,68 indicate that endplate facture triggers a sterile immune response. IL-8 and GROα attract neutrophils, MCP-1 attracts monocytes and macrophages, MCP-2 granulocytes, monocytes, T-cells, and NK cells, and MIG attracts T-cells. The release of inflammatory mediators and chemokines after tissue injury is inherent to almost all tissues and is required for tissue repair. However, a persistent injury stimulus as occurs with irreparable endplate fractures facilitates the formation of a destructive chronic inflammation.69 Once chronic inflammation occurs, resolution of inflammation and tissue healing are impeded. In contrast, no or reduced loading after endplate fracture in a rabbit explant model allowed resolution of the inflammation.26,70
The size of the endplate defect is critical for the severity of DD, because larger defects lead to more NP loss, stronger NP depressurization and more severe AF collapse.13,71,72 In small defects, where initial damage is limited, posttraumatic loading aggravates DD, because it maintains a high level of inflammation and catabolism.13
Another hallmark of post-traumatic DD is the over-expression of matrix-catabolic enzymes of the matrix metalloproteinases family (MMPs), especially of MMP1, -3, -13, which are all collagenases.69,73 MMPs not only cleave extracellular matrix substrates but also pre-cursors or active forms of cytokines.74 For example, MMP1, -3, and -13 cleave the N-terminus of MCP-1 and MCP-2 and transform their chemokine activity into an antagonistic activity and MMP1 and -3 degrade the active form of IL-1.74 On the other hand, MMP1 and MMP3 process proTNF into the active form. Therefore, MMPs have an important function in regulating the immune response.
Traumatic endplate damage also causes necrotic cell death13 and the release of intracellular contents, including many pro-inflammatory compounds known as damage-associated molecular patterns (DAMPs).75 DAMPs signal through toll-like receptors (TLRs) and cause inflammation. The activity of MMPs generates soluble and small extracellular-matrix fragments (e.g., biglycan, decorin, versican, fragments of fibronectin, hyaluronan), which can act as DAMPs. For example, the 30 kDa N-terminal fibronectin fragment is most abundant in degenerating discs of grade 3 and 4 and aggravates DD.76,77 In summary, disc cells respond to altered loads and osmotic pressures, to DAMPs, MMPs and pro-inflammatory cytokines with the expression of catabolic enzymes, with anabolic healing attempts, and with inflammation resulting in failed healing attempts, damage accumulation and chronic inflammation leading eventually to DD.
Endplate fractures compromise the disc immune privilege
The intervertebral disc is an immune privileged organ, because it is secluded from immune surveillance. The immune privilege is maintained by the expression of Fas ligand (FasL) on the surface of disc cells, which induces apoptosis in cells carrying the Fas receptor.78 Fas is expressed on most immune cells, including T-, B-, NK cells, macrophages, monocytes and granulocytes.
When the immune privilege is compromised (e.g., endplate fracture) or the disc is degenerated, disc cells start to express Fas receptor and transiently up-regulate FasL expression.79,80 The co-expression of FasL/Fas leads to an increased apoptosis rate through the type-II mitochondrial pathway.81,82 Eventually, FasL expression in disc cell decreases with degeneration83 and allows infiltration of immune cells, which are actively attracted by degenerating disc cells and add to the inflammatory milieu and DD.68,70
Reaction of the bone marrow to endplate defects
The physical breakage of the barrier between the disc and the vertebra with endplate fracture enhances fluid flow between the disc and the bone marrow.84 Fluids from the disc draining into the bone marrow likely carry cytokines, chemokines, MMPs and MMP-cleaved matrix catabolites and trigger pro-fibrotic marrow changes and an inflammatory dysmyelopoiesis (Fig. 7-5).85 Pro-inflammatory and pro-fibrotic disc / bone marrow cross-talk has also been demonstrated in patients with Modic changes.85 Modic changes are associated with endplate damage and disc degeneration.86,87 Modic change-like changes were also reported after perforation of the endplates in a pig model: oedema, fibrosis, and inflammatory cell infiltration into the bone marrow around the endplate defect were seen.88
Vice-versa, cytokines from bone marrow and bone cells also infiltrate the disc more easily with endplate damage. In experimental endplate trauma of rabbit spinal explant, co-cultured leukocytes did not infiltrate the disc but aggravated DD through up-regulation of MMP-1, MMP-3, TNF-α, IL-1, and IL-6. Therefore, leukocytes are important in posttraumatic DD even if they do not infiltrate the disc. Although Modic changes associate with endplate damage, they cannot be directly linked to traumatic events. Traumatic endplate fractures may cause a transient bone marrow oedema but chronic degenerative disc changes are required to trigger Modic changes.85,89 Yet, endplate fracture promote DD8 (Fig. 7-6).
Destructive Intervertebral Disc Injury (Organ Disruption), Disc Injury Type C
In this group the intervertebral disc is virtually destroyed by traumatic tear of the disc organ from the endplate as in some AO type B and C injuries.3,90 The annular fibers are stretched beyond their limits with a distractive or translational/rotational force direction. These injuries are highly unstable, and patients usually are undergoing surgery with some kind of spinal stabilization technique.91 Therefore, clinical studies on conservative treatment are lacking, and, likewise, the natural course is largely unknown. Only in the rare case of occult injuries or from case reports we can gain some insight.
Conceptually, the disruptive disc injuries can be sub-grouped into incomplete and complete dislocations. While in complete segmental separations all annular and nuclear fibers that are attached to the endplates must be torn; in incomplete dislocation, some fibers may remain intact.
Clinical studies disc injury type C
Typical injuries for this category are facet dislocations for the cervical36 and thoracolumbar spine92 or traumatic spondylolisthesis or retrolisthesis, especially at lumbocascral junction.93 For the lumbar and thoracic spine, Chance fractures94 were described as bony or soft tissue disruption resulting from a flexion distraction injury mechanism that occurs typically in passengers restrained by seat belts in traffic accidents. In a so-called soft tissue Chance fracture, typically the intervertebral disc and the facet joints, including the ligamentous complex, are disrupted.95 This reflects a gross segmental instability that is unlikely to heal as shown in a case report where secondary dislocation occurred after 4 weeks.96
Experimental studies disc injury type C
There are currently no biological studies that investigate complete destructive disc injuries as in fracture dislocations. Biomechanical studies demonstrated for the cervical spine that a complete cervical facet dislocation required an axial vertebral separation of 5.3 mm, a flexion angle of 63.1 degrees and shear displacement of 21.5 mm.97 A unilateral facet dislocation is likewise leading to complete disc destruction as after relocation of the facet (unlocking) a substantial segmental instability was observed.36
SUMMARY
Spine trauma can lead to a measurable damage up to a complete destruction of the intervertebral disc. The consequences of intervertebral disc and endplate trauma have been well studied in clinical as well as experimental, biological and biomechanical investigations. The posttraumatic changes and biological response is a multifactorial process, which demonstrates characteristics of idiopathic disc degeneration. However, similar to the susceptibility to idiopathic disc degeneration, spine trauma does not cause posttraumatic degeneration and loss of disc function in every patient even in the presence of an endplate fracture.6,98 The reason for this observation is not clear. It may be due to a superior regenerative potential (repair) and/or certain resilience to mechanical influences.
Further studies are needed to find the proper treatment for the spine trauma patient. If in stable fractures the intervertebral disc can be retained, it would be necessary to assess the individual risk factors for a degenerative course and to elucidate the value of temporary instrumentation and stress-shielding of the disc after trauma as well as of posttraumatic loading.
REFERENCES
- Battie MC, Videman T, Gill K, et al. 1991 Volvo Award in clinical sciences. Smoking and lumbar intervertebral disc degeneration: an MRI study of identical twins. Spine (Phila Pa 1976). 1991;16(9):1015-1021.
- Battie MC, Videman T. Lumbar disc degeneration: epidemiology and genetics. J Bone Joint Surg Am. 2006;88 Suppl 2:3-9.
- Schnake KJ, Schroeder GD, Vaccaro AR, Oner C. AOSpine Classification Systems (Subaxial, Thoracolumbar). J Orthop Trauma. 2017;31 Suppl 4:S14-S23.
- Schneiderman G, Flannigan B, Kingston S, Thomas J, Dillin WH, Watkins RG. Magnetic resonance imaging in the diagnosis of disc degeneration: correlation with discography. Spine (Phila Pa 1976). 1987;12(3):276-281.
- Luo L, Zhang C, Zhou Q, et al. Effectiveness of transpedicular dynamic stabilization in treating discogenic low back pain. World Neurosurg. 2018.;111:e192-e198.
- Furderer S, Wenda K, Thiem N, Hachenberger R, Eysel P. Traumatic intervertebral disc lesion--magnetic resonance imaging as a criterion for or against intervertebral fusion. Eur Spine J. 2001;10(2):154-163.
- Sander AL, Lehnert T, El Saman A, Eichler K, Marzi I, Laurer H. Outcome of traumatic intervertebral disk lesions after stabilization by internal fixator. AJR Am J Roentgenol. 2014;203(1):140-145.
- Sander AL, Laurer H, Lehnert T, et al. A clinically useful classification of traumatic intervertebral disk lesions. AJR Am J Roentgenol. 2013;200(3):618-623.
- Daly C, Ghosh P, Jenkin G, Oehme D, Goldschlager T. A review of animal models of intervertebral disc degeneration: pathophysiology, regeneration, and translation to the clinic. Biomed Res Int. 2016;2016:5952165.
- Jin L, Balian G, Li XJ. Animal models for disc degeneration-an update. Histol Histopathol. 2018;33(6):543-554.
- Singh K, Masuda K, An HS. Animal models for human disc degeneration. Spine J. 2005;5(6 Suppl):267S-279S.
- Gantenbein B, Illien-Junger S, Chan SC, et al. Organ culture bioreactors--platforms to study human intervertebral disc degeneration and regenerative therapy. Curr Stem Cell Res Ther. 2015;10(4):339-352.
- Dudli S, Ferguson SJ, Haschtmann D. Severity and pattern of post-traumatic intervertebral disc degeneration depend on the type of injury. Spine J. 2014;14(7):1256-1264.
- Haschtmann D, Stoyanov JV, Gedet P, Ferguson SJ. Vertebral endplate trauma induces disc cell apoptosis and promotes organ degeneration in vitro. Eur Spine J. 2008;17(2):289-299.
- Lee CR, Iatridis JC, Poveda L, Alini M. In vitro organ culture of the bovine intervertebral disc: effects of vertebral endplate and potential for mechanobiology studies. Spine (Phila Pa 1976). 2006;31(5):515-522.
- Alini M, Eisenstein SM, Ito K, et al. Are animal models useful for studying human disc disorders/degeneration? Eur Spine J. 2008;17(1):2-19.
- Chan SC, Ferguson SJ, Gantenbein-Ritter B. The effects of dynamic loading on the intervertebral disc. Eur Spine J. 2011;20(11):1796-1812.
- Hancock MJ, Battie MC, Videman T, Gibbons L. The role of back injury or trauma in lumbar disc degeneration: an exposure-discordant twin study. Spine (Phila Pa 1976). 2010;35(21):1925-1929.
- Joaquim AF, Patel AA, Vaccaro AR. Cervical injuries scored according to the subaxial injury classification system: an analysis of the literature. J Craniovertebr Junction Spine. 2014;5(2):65-70.
- Curatolo M, Bogduk N, Ivancic PC, McLean SA, Siegmund GP, Winkelstein BA. The role of tissue damage in whiplash-associated disorders: discussion paper 1. Spine (Phila Pa 1976). 2011;36(25 Suppl):S309-315.
- Elliott JM, Dayanidhi S, Hazle C, et al. Advancements in imaging technology: do they (or will they) equate to advancements in our knowledge of recovery in whiplash? J Orthop Sports Phys Ther. 2016;46(10):862-873.
- Teraguchi M, Yoshimura N, Hashizume H, et al. Prevalence and distribution of intervertebral disc degeneration over the entire spine in a population-based cohort: the Wakayama Spine Study. Osteoarthritis Cartilage. 2014;22(1):104-110.
- Matsumoto M, Ichihara D, Okada E, et al. Modic changes of the cervical spine in patients with whiplash injury: a prospective 11-year follow-up study. Injury. 2013;44(6):819-824.
- Kerttula LI, Serlo WS, Tervonen OA, Paakko EL, Vanharanta HV. Post-traumatic findings of the spine after earlier vertebral fracture in young patients: clinical and MRI study. Spine (Phila Pa 1976). 2000;25(9):1104-1108.
- MacLean JJ, Roughley PJ, Monsey RD, Alini M, Iatridis JC. In vivo intervertebral disc remodeling: kinetics of mRNA expression in response to a single loading event. J Orthop Res. 2008;26(5):579-588.
- Dudli S, Haschtmann D, Ferguson SJ. Fracture of the vertebral endplates, but not equienergetic impact load, promotes disc degeneration in vitro. J Orthop Res. 2012;30(5):809-816.
- Gallagher S, Marras WS, Litsky AS, Burr D. Torso flexion loads and the fatigue failure of human lumbosacral motion segments. Spine (Phila Pa 1976). 2005;30(20):2265-2273.
- Curry WH, Pintar FA, Doan NB, et al. Lumbar spine endplate fractures: biomechanical evaluation and clinical considerations through experimental induction of injury. J Orthop Res. 2016;34(6):1084-1091.
- Wang JL, Panjabi MM, Kato Y, Nguyen C. Radiography cannot examine disc injuries secondary to burst fracture: quantitative discomanometry validation. Spine (Phila Pa 1976). 2002;27(3):235-240.
- Graham J, Ahn C, Hai N, Buch BD. Effect of bone density on vertebral strength and stiffness after percutaneous vertebroplasty. Spine (Phila Pa 1976). 2007;32(18):E505-511.
- Barak MM, Weiner S, Shahar R. The contribution of trabecular bone to the stiffness and strength of rat lumbar vertebrae. Spine (Phila Pa 1976). 2010;35(22):E1153-1159.
- Hulme PA, Boyd SK, Ferguson SJ. Regional variation in vertebral bone morphology and its contribution to vertebral fracture strength. Bone. 2007;41(6):946-957.
- Legrand E, Chappard D, Pascaretti C, et al. Trabecular bone microarchitecture, bone mineral density, and vertebral fractures in male osteoporosis. J Bone Miner Res. 2000;15(1):13-19.
- Jackman TM, Hussein AI, Curtiss C, et al. Quantitative, 3D visualization of the initiation and progression of vertebral fractures under compression and anterior flexion. J Bone Miner Res. 2016;31(4):777-788.
- Kiehl KL, Curry WH, Stemper BD, et al. A method for inducing and determining biomechanics associated with endplate fractures in the lumbar spine. Biomed Sci Instrum. 2014;50:119-124.
- Crawford NR, Duggal N, Chamberlain RH, Park SC, Sonntag VK, Dickman CA. Unilateral cervical facet dislocation: injury mechanism and biomechanical consequences. Spine (Phila Pa 1976). 2002;27(17):1858-1864; discussion 1864.
- Dundamadappa SK, Cauley KA. MR imaging of acute cervical spinal ligamentous and soft tissue trauma. Emerg Radiol. 2012;19(4):277-286.
- Schwarz N, Genelin F, Schwarz AF. Post-traumatic cervical kyphosis in children cannot be prevented by non-operative methods. Injury. 1994;25(3):173-175.
- Wade KR, Robertson PA, Thambyah A, Broom ND. How healthy discs herniate: a biomechanical and microstructural study investigating the combined effects of compression rate and flexion. Spine (Phila Pa 1976). 2014;39(13):1018-1028.
- Carragee EJ, Don AS, Hurwitz EL, Cuellar JM, Carrino JA, Herzog R. 2009 ISSLS Prize Winner: Does discography cause accelerated progression of degeneration changes in the lumbar disc: a ten-year matched cohort study. Spine (Phila Pa 1976). 2009;34(21):2338-2345.
- Rajasekaran S, Bajaj N, Tubaki V, Kanna RM, Shetty AP. ISSLS Prize winner: The anatomy of failure in lumbar disc herniation: an in vivo, multimodal, prospective study of 181 subjects. Spine (Phila Pa 1976). 2013;38(17):1491-1500.
- Kadam G, Narsinghpura K, Deshmukh S, Desai S. Traumatic lumbar vertebral ring apophysis fracture with disk herniation in an adolescent. Radiol Case Rep. 2017;12(2):427-430.
- Ikata T, Morita T, Katoh S, Tachibana K, Maoka H. Lesions of the lumbar posterior end plate in children and adolescents. An MRI study. J Bone Joint Surgery Br. 1995;77(6):951-955.
- Takata K, Inoue S, Takahashi K, Ohtsuka Y. Fracture of the posterior margin of a lumbar vertebral body. J Bone Joint Surg Am. 1988;70(4):589-594.
- Snow CR, Harvey-Burgess M, Laird B, Brown SHM, Gregory DE. Pressure-induced end-plate fracture in the porcine spine: is the annulus fibrosus susceptible to damage? Eur Spine J. 2018;27(8):1767-1774.
- Adams MA. Biomechanics of back pain. Acupunct Med. 2004;22(4):178-188.
- Ishihara H, McNally DS, Urban JP, Hall AC. Effects of hydrostatic pressure on matrix synthesis in different regions of the intervertebral disk. J Appl Physiol (1985). 1996;80(3):839-846.
- Dolan P, Luo J, Pollintine P, Landham PR, Stefanakis M, Adams MA. Intervertebral disc decompression following endplate damage: implications for disc degeneration depend on spinal level and age. Spine (Phila Pa 1976). 2013;38(17):1473-1481.
- Adams MA, Dolan P. Intervertebral disc degeneration: evidence for two distinct phenotypes. J Anat. 2012;221(6):497-506.
- Le Maitre CL, Richardson SM, Baird P, Freemont AJ, Hoyland JA. Expression of receptors for putative anabolic growth factors in human intervertebral disc: implications for repair and regeneration of the disc. J Pathol. 2005;207(4):445-452.
- Masuda K, Aota Y, Muehleman C, et al. A novel rabbit model of mild, reproducible disc degeneration by an anulus needle puncture: correlation between the degree of disc injury and radiological and histological appearances of disc degeneration. Spine (Phila Pa 1976). 2005;30(1):5-14.
- Ulrich JA, Liebenberg EC, Thuillier DU, Lotz JC. ISSLS prize winner: repeated disc injury causes persistent inflammation. Spine (Phila Pa 1976). 2007;32(25):2812-2819.
- Virri J, Grönblad M, Seitsalo S, Habtemariam A, Kääpä E, Karaharju E. Comparison of the prevalence of inflammatory cells in subtypes of disc herniations and associations with straight leg raising. Spine (Phila Pa 1976). 2001;26(21):2311-2315.
- Takada T, Nishida K, Maeno K, et al. Intervertebral disc and macrophage interaction induces mechanical hyperalgesia and cytokine production in a herniated disc model in rats. Arthritis Rheum. 2012;64(8):2601-2610.
- Finskas O, Blixt A, Fujioka Y, Olmarker K. New, clinically more relevant model for nerve root injury in the rat. Spine. 2013;38(20):1744-1748.
- Geiss A, Larsson K, Junevik K, Rydevik B, Olmarker K. Autologous nucleus pulposus primes T cells to develop into interleukin-4-producing effector cells: an experimental study on the autoimmune properties of nucleus pulposus. J Orthop Res. 2009;27(1):97-103.
- Stirling A, Worthington T, Rafiq M, Lambert PA, Elliott TS. Association between sciatica and Propionibacterium acnes. Lancet. 2001;357(9273):2024-2025.
- Albert HB, Lambert P, Rollason J, et al. Does nuclear tissue infected with bacteria following disc herniations lead to Modic changes in the adjacent vertebrae? Eur Spine J. 2013;22(4):690-696.
- Dudli S, Liebenberg E, Magnitsky S, Miller S, Demir-Deviren S, Lotz JC. Propionibacterium acnes infected intervertebral discs cause vertebral bone marrow lesions consistent with Modic changes. J Ortho Res. 2016;34(8):1447-1455.
- Dudli S, Miller S, Demir-Deviren S, Lotz JC. Inflammatory response of disc cells against Propionibacterium acnes depends on the presence of lumbar Modic changes. Eur Spine J. 2018;27(5):1013-1020.
- Möller A, Maly P, Besjakov J, Hasserius R, Ohlin A, Karlsson MK. A vertebral fracture in childhood is not a risk factor for disc degeneration but for Schmorl’s nodes: a mean 40-year observational study. Spine (Phila Pa 1976). 2007;32(22):2487-2492.
- Vornanen M, Böstman O, Keto P, Myllynen P. The integrity of intervertebral disks after operative treatment of thoracolumbar fractures. Clin Ortho Relat Res. 1993(297):150-154.
- Oner FC, van der Rijt RR, Ramos LM, Dhert WJ, Verbout AJ. Changes in the disc space after fractures of the thoracolumbar spine. J Bone Joint Surg Br. 1998;80(5):833-839.
- Hsu K, Zucherman J, Shea W, et al. High lumbar disc degeneration. Incidence and etiology. Spine (Phila Pa 1976). 1990;15(7):679-682.
- Mok FP, Samartzis D, Karppinen J, Luk KD, Fong DY, Cheung KM. ISSLS prize winner: prevalence, determinants, and association of Schmorl nodes of the lumbar spine with disc degeneration: a population-based study of 2449 individuals. Spine (Phila Pa 1976). 2010;35(21):1944-1952.
- Kyere KA, Than KD, Wang AC, et al. Schmorl’s nodes. Eur Spine J. 2012;21(11):2115-2121.
- Holm S, Baranto A, Kaigle Holm A, et al. Reactive changes in the adolescent porcine spine with disc degeneration due to endplate injury. Vet Comp Ortho Traumatol. 2007;20(1):12-17.
- Alkhatib B, Rosenzweig DH, Krock E, et al. Acute mechanical injury of the human intervertebral disc: link to degeneration and pain. Eur Cells Mater. 2014;28:98-110; discussion 110-111.
- Dudli S, Haschtmann D, Ferguson SJ. Persistent degenerative changes in the intervertebral disc after burst fracture in an in vitro model mimicking physiological post-traumatic conditions. Eur Spine J. 2015;24(9):1901-1908.
- Dudli S, Boffa DB, Ferguson SJ, Haschtmann D. Leukocytes enhance inflammatory and catabolic degenerative changes in the intervertebral disc after endplate fracture in vitro without infiltrating the disc. Spine (Phila Pa Spine). 2015;40(23):1799-1806.
- Sitte I, Klosterhuber M, Lindtner RA, et al. Morphological changes in the human cervical intervertebral disc post trauma: response to fracture-type and degeneration grade over time. Eur Spine J. 2016;25(1):80-95.
- Zehra U, Flower L, Robson-Brown K, Adams MA, Dolan P. Defects of the vertebral end plate: implications for disc degeneration depend on size. Spine J. 2017;17(5):727-737.
- Weiler C, Nerlich AG, Zipperer J, Bachmeier BE, Boos N. 2002 SSE Award Competition in Basic Science: expression of major matrix metalloproteinases is associated with intervertebral disc degradation and resorption. Eur Spine J. 2002;11(4):308-320.
- Parks WC, Wilson CL, Lopez-Boado YS. Matrix metalloproteinases as modulators of inflammation and innate immunity. Nat Rev Immunol. 2004;4(8):617-629.
- Piccinini AM, Midwood KS. DAMPening inflammation by modulating TLR signalling. Mediators Inflamm. 2010;2010.
- Ruel N, Markova DZ, Adams SL, et al. Fibronectin fragments and the cleaving enzyme ADAM-8 in the degenerative human intervertebral disc. Spine (Phila Pa 1976). 2014;39(16):1274-1279.
- Greg Anderson D, Li X, Tannoury T, Beck G, Balian G. A fibronectin fragment stimulates intervertebral disc degeneration in vivo. Spine (Phila Pa 1976). 2003;28(20):2338-2345.
- Liu ZH, Sun Z, Wang HQ, et al. FasL expression on human nucleus pulposus cells contributes to the immune privilege of intervertebral disc by interacting with immunocytes. Int J Med Sci. 2013;10(8):1053-1060.
- Wang J, Tang T, Yang H, et al. The expression of Fas ligand on normal and stabbed-disc cells in a rabbit model of intervertebral disc degeneration: a possible pathogenesis. J Neurosurg Spine. 2007;6(5):425-430.
- Haschtmann D, Ferguson SJ, Stoyanov JV. Apoptosis and gene expression of collagenases but not gelatinases in rabbit disc fragment cultures. J Neurosurg Spine. 2008;8(6):552-560.
- Heyde CE, Tschoeke SK, Hellmuth M, Hostmann A, Ertel W, Oberholzer A. Trauma induces apoptosis in human thoracolumbar intervertebral discs. BMC Clin Pathol. 2006;6:5.
- Park JB, Lee JK, Park SJ, Kim KW, Riew KD. Mitochondrial involvement in fas-mediated apoptosis of human lumbar disc cells. J Bone Joint Surgery Am. 2005;87(6):1338-1342.
- Kaneyama S, Nishida K, Takada T, et al. Fas ligand expression on human nucleus pulposus cells decreases with disc degeneration processes. J Ortho Sci. 2008;13(2):130-135.
- Rajasekaran S, Babu JN, Arun R, Armstrong BR, Shetty AP, Murugan S. ISSLS prize winner: A study of diffusion in human lumbar discs: a serial magnetic resonance imaging study documenting the influence of the endplate on diffusion in normal and degenerate discs. Spine (Phila Pa 1976). 2004;29(23):2654-2667.
- Dudli S, Sing DC, Hu SS, et al. ISSLS Prize in Basic Science 2017: Intervertebral disc/bone marrow cross-talk with Modic changes. Eur Spine J. 2017;26(5):1362-1373.
- Weiner BK, Vilendecic M, Ledic D, et al. Endplate changes following discectomy: natural history and associations between imaging and clinical data. Eur Spine J. 2015;24(11):2449-2457.
- Farshad-Amacker NA, Hughes A, Herzog RJ, Seifert B, Farshad M. The intervertebral disc, the endplates and the vertebral bone marrow as a unit in the process of degeneration. Eur Radiology. 2017;27(6):2507-2520.
- Holm S, Mackiewicz Z, Holm AK, et al. Pro-inflammatory, pleiotropic, and anti-inflammatory TNF-alpha, IL-6, and IL-10 in experimental porcine intervertebral disk degeneration. Vet Pathol. 2009;46(6):1292-1300.
- Torkki M, Majuri ML, Wolff H, et al. Osteoclast activators are elevated in intervertebral disks with Modic changes among patients operated for herniated nucleus pulposus. Eur Spine J. 2016;25(1):207-216.
- Vaccaro AR, Koerner JD, Radcliff KE, et al. AOSpine subaxial cervical spine injury classification system. Eur Spine J. 2016;25(7):2173-2184.
- Haschtmann D, Stahel PF, Heyde CE. Management of a multiple trauma patient with extensive instability of the lumbar spine as a result of a bilateral facet dislocation and multiple complete vertebral burst fractures. J Trauma. 2009;66(3):922-930.
- Levine AM, Bosse M, Edwards CC. Bilateral facet dislocations in the thoracolumbar spine. Spine (Phila Pa 1976). 1988;13(6):630-640.
- Pourabbas B, Effani MA, Namdari A. Traumatic retrolisthesis of L5 and L5/S1 extruded disc herniation; a case report and review of the literature. Bull Emerg Trauma. 2016;4(3):170-173.
- Chance GQ. Note on a type of flexion fracture of the spine. Br J Radiol. 1948;21(249):452.
- Smith WS, Kaufer H. Patterns and mechanisms of lumbar injuries associated with lap seat belts. J Bone Joint Surg Am. 1969;51(2):239-254.
- Bourne JT, Baker ADL, Khatri M. A combined bony and soft tissue, thoracic chance fracture: late displacement following conservative treatment. Case Rep Orthop. 2017;2017:6528673.
- Ivancic PC, Pearson AM, Tominaga Y, Simpson AK, Yue JJ, Panjabi MM. Biomechanics of cervical facet dislocation. Traffic Inj Prev. 2008;9(6):606-611.
- Alanay A, Yazici M, Acaroglu E, Turhan E, Cila A, Surat A. Course of nonsurgical management of burst fractures with intact posterior ligamentous complex: an MRI study. Spine Phila Pa 1976). 2004;29(21):2425-2431.