Lydia Jones and R. Shane Tubbs
INTRODUCTION
As with the other regions of the spine, the lumbar vertebrae rely on a robust collection of soft tissues to maintain their structural integrity during load bearing and movement. Of these soft tissues, the lumbar vertebral ligaments play a very important role. These highly specialized structures allow for extremes of movement while maintaining alignment of the spinal canal, intervertebral foramina and intervertebral discs. Therefore, disruption of these structures can lead to significant neurological compromise.
ANTERIOR LONGITUDINAL LIGAMENT
Anatomy
The anterior longitudinal ligament (ALL) (Fig. 2-1) is composed of a strong band of collagenous fibers that extends along the anterior aspect of the vertebral column.1,2,3,4 The ALL is attached to the basilar occipital bone rostrally, extends to the anterior tubercle of the atlas (C1) as well as the front of the body of the axis (C2), and runs caudally to the anterior of the sacrum.1 The ALL is narrower and thicker over the vertebral bodies than when it passes over the intervertebral (IV) discs.1,3 Likewise, it is also thicker and narrower in the thoracic region, as compared to its presence in the cervical and lumbar regions, and broadens as it travels caudally.1 The average width of the ALL in the lumbar spine in 25 cadaveric specimens was measured to be 37.1 mm with a mean cross-sectional area of 32.4 mm2 and a range from 10.6–52.5 mm2.5
The ALL consists of numerous collagen fibers that are densely packed and constitute three distinct layers: superficial, intermediate, and deep.1,2,4 The superficial fibers are the longest, spanning anywhere from three to five vertebrae, while the intermediate fibers span between two to three vertebrae, and the deep fibers span from one vertebral body to the next, connecting adjacent vertebrae.1,4 These longitudinal fibers attach strongly to the IV discs and the anterior margins of the vertebral bodies.1,2,3 These fibers form looser, secondary attachments to the mid-portion of the vertebral bodies, filling their concave surfaces and flattening the appearance of anterior surface of the vertebral column.2 The deep fibers of the ALL blend with the periosteum at this point, while the more superficial fibers bridge the concavity.2 The fibers from the ALL also blend with the external surface of the annulus fibrosus, which has led anatomists to varying opinions on the origins of these fibers.2 While some authors believe these fibers to be part of the annulus fibrosus and others feel they constitute a ‘disc capsule,’ Bogduk demonstrated that the deep fibers of the ALL should not be considered part of the annulus fibrosus due to differences in the embryological origin of these two structures.2 Accordingly, the fibers of the ALL have attachments to cortical bone, which is always the case with ligaments.2 In 1997, Bogduk demonstrated that the annulus fibrosus does not attach to cortical bone but rather to the vertebral end plate.2 Bogduk also illustrated some key differences in ALL structure at varying regions of the vertebral column such as the thoracic region, which was the only location where the ALL was not associated with any of the prevertebral muscles and therefore, stood alone.2 Another distinction was made in the lumbar spine at the point where the crura of the diaphragm attached to the first three lumbar vertebrae.2 In this region, the tendons of the crura extend caudally beyond the three vertebrae and form much of what is described as the ALL in this region.2 This peculiarity has led Bogduk to suggest that the ALL may, to some extent, be a continuation of a tendon attachment in addition to its ligamentous nature.2
Biomechanics
The ALL primarily functions to maintain the stability of the vertebral column by restricting the motion of the spinal segments during extension.2,6 While Neumann et al., in 1992, suggested that the ALL functions during extension, lateral bending, and axial rotation, Zander et al., in 2004, believed that the ALL is only loaded during extension and is unloaded during other movements of the spinal column.7,8 The ALL is the strongest and largest of the spinal ligaments and is the only one that limits extension; the remaining IV ligaments limit flexion movements.6,7,8 In a study conducted by Neumann et al. in 1992, they found the stiffness of the ALL to be significantly greater than that of the posterior spinal ligaments.7 They also noted that the ALL strength decreased slightly in subjects between the ages of 21 to 43 years.7 In a subsequent study in 1993, Neumann et al. found the mean failure loads of bone-ALL-bone complexes to be 802 Newtons (N).9 This result is drastically different from other studies, in which the mean values ranged from 330–473 N.9 Neumann and colleagues explained this difference by the properties of the specimens used in their study; they suggested that the difference was due to using specimens from younger and, presumably, less sedentary specimens, whereas other studies used ALL specimens from older subjects.9 They also found that younger ALL specimens were more than twice as strong as older specimens.9 They proposed, however, that bone mineral content is a more accurate predictor of bone-ligament-bone structural properties than is age, as there is a linear increase in ultimate force, stiffness, and energy of this complex with increasing bone density.9 In 1992, Pintar et al. reported the mean stiffness value for the ALL in the lumbar spine to be 33.0 N/mm with a margin of 15.7 N/mm.5 The highest values of energy to failure were observed for the ALL and the supraspinous ligament (SSL), with values ranging from 0.82–8.68 Joules (J) for the ALL and 3.18–11.64 J for the SSL.5 According to Panjabi et al. in 1982, failure results from these ligaments needing to withstand functionally more force and deformation.10 Failure stresses were found to be similar between the ALL and posterior longitudinal ligament (PLL), at 8.2–16.1 MPa and 7.2–28.4 MPa, respectively.5 The values of stress and strain at failure reported by Pintar et al. in 1992 were similar to the values reported by Chazal et al. in 1985.5,11
POSTERIOR LONGITUDINAL LIGAMENT
Anatomy
The posterior longitudinal ligament (PLL) (Fig. 2-2) is a band of longitudinal fibers that runs within the vertebral canal along the posterior aspect of the vertebral bodies.1,2,3,4 Rostrally, the PLL is continuous with the tectorial membrane, and in the upper lumbar region the PLL takes on a caudal direction. Attaching to the tip of the vertebral body below, the lateral deep fibers at this level take a horizontal path and extend out to the IV foramen, covering the lower half of the annulus fibrosus and attaching at its lateral opening.4 The average width of the PLL in the lumbar spines of 21 cadaveric specimens was 33.3 mm, with a mean cross-sectional area of 5.2 mm2.5
The fibers of the PLL are more compact than those of the ALL.3 Between the ligamentous fibers lay the basivertebral veins and the venous rami that drain them into anterior internal vertebral plexuses.1 Early studies also describe an abundance of free and encapsulated nerve endings within the PLL.4 Similar to the ALL, the superficial fibers of the PLL can span anywhere from three to five vertebrae and the deepest fibers are between adjacent vertebrae.1,2 These layers, however, are more pronounced in the immediate postnatal years.1 Examination of the layers of the PLL showed that the superficial fibers measure a length of 0.5–1.0 cm.4 The fibers of the PLL attach more firmly to the IV discs than the vertebral bodies, with deep fibers fused to the annuli fibrosi in the adult spine and the superficial fibers being more easily separable from the deeper fibers when over the vertebral bodies.1,4 The deep layer of the PLL forms a narrow ligament at the middle of the vertebral body, but as it travels caudally and approaches the upper edge of the IV space, it diverges towards the lip of the next lower vertebral body, as well as a portion of the pedicle of that next lower vertebral body.4 As per Bogduk, the deep fibers of the PLL not only fuse with the fibers of the annulus fibrosus, but ultimately penetrate through them to attach to the posterior margins of the vertebral bodies.2 In this way, these deep fibers act much like ‘paravertebral ligaments.’1
Biomechanics
The PLL functions to resist hyperflexion of the vertebral column by resisting separation of the posterior ends of the vertebral bodies.2,6 However, due to the polysegmental nature of this ligament, its force is applied over several inter-body joints as opposed to just one.2 The PLL also functions to prevent posterior herniation of the nucleus pulposus.6 It contains nociceptive nerve endings and is the source of pain from IV disc herniation as the annulus fibrosus impinges on the ligament.6 Pintar et al. reported that the PLL has the smallest cross-sectional area, with a mean of 5.2 mm2, compared to the other lumbar spinal ligaments.5 This ligament also had the lowest value of energy to failure, at a range of 0.07–0.33 J, as well as the least strain at failure, ranging between 11.3–16.2 percent.5 Failure stresses were found to be similar between the ALL and PLL at 8.2–16.1 MPa and 7.2–28.4 MPa, respectively.5 Similar values for stress and strain at failure were reported by Chazal et al. in 1985.5,11 The mean stiffness value for the PLL in the lumbar spine was 20.4 ± 11.9 Nmm-1.5
LIGAMENTUM FLAVUM
Anatomy
The ligamenta flava (LF) (Fig. 2-3) are a series of ligaments that connect the lamina of adjacent vertebrae to form alternating sections of the posterior wall of the vertebral canal.1,2,3,6 The LF are present bilaterally at each vertebral level, converging in the midline.2,6 This ligament’s perpendicular fibers attach to the anterior surface of the lower edge of the lamina above, as well as the inferior aspect of the pedicle, and descend to the posterior surface of the upper edge of the lamina below.1,2,3,6,12 As this ligament descends inferiorly, it divides into medial and lateral portions.2 The medial portion passes to the back of the next lower lamina to attach to the rough area on the upper quarter of the dorsal surface of the lamina, and the lateral portion passes in front of the zygapophysial joint formed by two vertebrae that the ligament connects.2 Inferiorly, the lateral portion of each ligament extends to the midpoint between two pedicles and forms the anterior capsule of the zygapophysial joint, as it attaches to the anterior aspects of the inferior and superior articular processes of the zygapophysial joint.1,2,12 In fact, the most lateral fibers extend along the root of the superior articular process as far as the next lower pedicle to which they are attached.2 From where the posterior margins of the ligament meet as the ligament’s attachments extend from the zygapophysial capsule to where the laminae fuse to form spines, the ligament is only partially united, with intervals being left for veins connecting the internal to posterior external posterior vertebral venous plexus.1 In 1992, Pintar and colleagues measured the lumbar LF in 22 cadaveric specimens and found that, on average, the LF measures 15.2 mm in length with a mean cross-sectional area of 84.2 mm2.5 In the cervical region, the LF are thin and broad, thickening as they descend caudally, with the thickest ligaments found in the lumbar region.1,6 The average thickness of the LF, both at midline and laterally, was noted to be 2–3 mm by Yong-Hing and colleagues in 1976.12
Biomechanics
The LF functions to oppose separation of the vertebral laminae during spinal flexion.1,2,3,6 This is accomplished by arresting abrupt flexion and aiding in restoring the vertebral column to an erect posture, thereby preserving the normal curvature of spine and protecting the IV discs from injury.1,2,3,6,13 The elastic nature of this ligament plays an important role in maintaining this function, as a collagenous ligament would be able to resist separation but would buckle when the laminae were approximated.2 On average, it is composed of 80% elastin and 20% collagen with the elastic fibers being oriented parallel to the long axis of the ligament.1,10,14 Yong-Hing and colleagues, in 1976, obtained 51 specimens, which were stained with Gomori’s aldehyde fuschin stain to measure the elastin (stained purple) and collagen (stained green) content of these ligaments.14 In these specimens, they found that the ligaments’ composition consisted of 50–80% elastin and 20–50% collagen.14 While anteriorly the ligament is thin due to the elastic fibers, the posterior capsule of each posterior joint is thicker due to the collagenous content.14 A study on the ultra-structure of the ligament demonstrated that the LF contains two morphologically different types of elastin fibers: elastic and elaunin fibers.10 While the elastic fibers proper were found throughout the ligament, though mainly in the central region, the elaunin fibers, which are composed of a few elastin deposits interspersed with microtubules, were more prominent along the bony attachments of the ligaments.1,10 Very few spindle-shaped fibroblasts were also associated with the ligament.10 The LF differs from all other ligaments of the lumbar spine, as it is the only ligament consisting of predominately elastic fibers as opposed to collagen.1 The LF are noted to retain their elastin content with increasing age.14 While it was previously postulated that the elastin content of the ligament decreases and the ligament becomes more rigid in patients with spondylosis, Yong-Hing and colleagues found no evidence to support this claim; instead, they noted that there is no correlation between elastin content and age, duration of symptoms of low back or leg pain, or the presence of degenerative spondylosis.14 They also noted that there was no evidence that, with increasing age, any hypertrophy, hyperplasia, or degeneration of elastin takes place.14
Buckling is minimal with an elastic ligament, protecting the contents of the spinal canal from damage.2 The elastic nature of this ligament aids in restoring a flexed lumbar spine to its extended position while the lateral division of the ligament will prevent the anterior capsule of the zygapophysial joint from being nipped within the joint capsule during movement.2 The LF exerts a disc pressure of 0.7 kg/cm2 and serves to pre-stress the IV disc; however, the biological significance of this is unknown.2 The lateral portion of the ligament prevents injury to the anterior capsule of the zygapophysial joint during movement.2 While some believe that the LF assists in extension of the spine with the help of the erector spinae muscles, Bogduk questions the importance of this ligament in producing extension movements.2,12 Pintar et al. reported that the LF exhibited the greatest cross-sectional area compared to the other lumbar spinal ligaments, with a mean area of 84.2 ± 17.9 mm2, similar to the values reported by Panjabi et al. in 1991.5,14 The LF had the lowest overall failure stresses at 1.3–4.1 MPa, with findings similar to those reported by Nachemson and Evans in 1968.5,15 Similar values for stress and strain at failure were reported by Chazal et al. in 1985.5,11 Panjabi et al., in 1991, reported the LF lengths as ranging from 11.6–16.0 mm, which is, again, similar to the range (13.0–18.0 mm) reported by Pintar et al. in 1992.5,14 The mean stiffness value for the LF was 27.2 ± 9.2 Nmm-1.5
There have been no reported disabilities in patients with ligamentum flava excised at single or multiple sites; this is most likely due to the location rather than the function of the ligament, as the ligament lies immediately behind the vertebral canal and is, therefore, immediately adjacent to nervous structures within the canal.2 The lateral extensions of the ligamentum flava have elasticity of medial and anterior capsule of the posterior joints that balances the elasticity of the intervertebral disc.12
SUPRASPINOUS LIGAMENT
Anatomy
The supraspinous ligament (SSL) (Figs. 2-4 and 2-5) is composed of a strong fibrous cord connecting the tips of spinous processes of the vertebral column.1,2,3,6 Fibrocartilage has been noted to be present where the ligament attaches to the spinous process.3 There are inconsistencies in the description of the SSL among anatomists, with many anatomists describing the SSL as beginning at the seventh cervical vertebra, where it then merges with the nuchal ligament to extend to the occipital protuberance, and extending in a caudal direction along the length of the vertebral column to the sacrum.1,3,6 As this ligament passes caudally, it will thicken and become broader in the lumbar region where it will blend intimately with the neighboring fascia.1 While there is no disagreement about where the ligament begins cranially, Parke, Heylings, and Bogduk all suggest that the SSL extends caudally only as far as the fifth lumbar vertebra.1,2,16,17 According to Bogduk, the SSL bridges the L4-L5 interspace in only 5% of individuals.2 He suggests that the ligament ends at the third lumbar spinous process in 22% of individuals and at L4 in the remaining 73%.2 Caudal to L5, the SSL is replaced by medial tendons of the erector spinae muscle.16 The average length of the SSL in the lumbar spine is 25.2 mm with a mean cross-sectional area of 25.2 mm2 based on a study of 22 cadaveric specimens.5
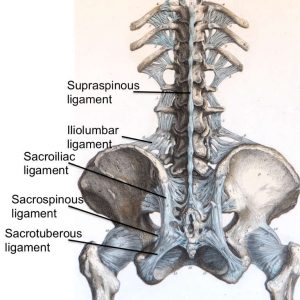
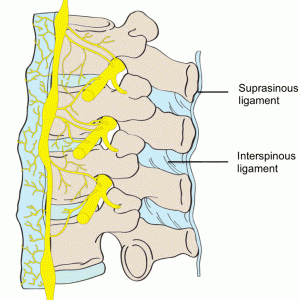
Biomechanics
The SSL functions to limit flexion but provides little resistance to separation of the spinous processes.2,16 Heylings suggests that the absence of the SSL at the lumbosacral junction may account for the increased mobility of the spine in this region.16 He also suggests that this absence may play a role in the etiology of low back pain and the musculoskeletal disorders that often affect the lumbosacral junction.16 In 1999, Gudavalli and Triano used computer modeling to assess the lumbar spinal ligaments and found that the SSL carries the greatest load during flexion, followed by the LF, capsular ligament, and the intertransverse and interspinous ligaments.18 They also found that the SSL undergoes the greatest increase in length.18 Hindle and colleagues, in 1990, found that the supraspinous and interspinous ligaments carried very little load during the first half of flexion but resisted a much greater load towards the end of flexion.19 They suggest that while these ligaments are useful in limiting passive flexion when acting in concert with other support structures, they lack much mechanical function when isolated.19 Due to its continuous nature, the SSL was found to have one of the greatest original lengths, next to the ALL and PLL, when compared to other spinal ligaments.5 The highest values of energy to failure were observed for the ALL and the SSL, with values ranging from 0.82–8.68 J and 3.18–11.64 J, respectively.5 Pintar et al. also reported the SSL as having the highest strain at failure (70.6–115.0 %).5 The mean stiffness value reported for the SLL was 23.7 ± 10.9 Nmm-1.5
INTERSPINOUS LIGAMENT
Anatomy
The interspinous ligament (ISL) (Fig. 2-5) is a thin and almost membranous ligament connecting adjacent vertebral spinous processes.1,2,3,6 Its attachments extend from the root to apex of each spinous process, meeting the SSL dorsally and the LF ventrally.1,3,6 The ISL is poorly developed in the neck, elongated and narrow in the thoracic region, and thicker and broader in the lumbar region.1,3 There are inconsistencies in the description of the fibers of the ISL.16 With regards to the cervical bundles, some anatomists describe these bundles of the ISL as part of the ligamentum nuchae, while others describe them as distinct interspinous fascicles.1 Also, major discrepancies exist regarding the lumbar supraspinous and interspinous ligaments in humans.16 Many anatomists describe these fibers as crossing the interspinous space in a posterocaudal direction.1,17,20 However, Heylings, Rissanen, Grant, and Bogduk describe the fibers as traversing in a posterocranial direction, especially in the lumbar spine where, anteriorly, the ISL is essentially a paired structure with ligaments on each side, split by a mid-line cavity filled with fat.2,16,21,22 This cavity, however, is not present more posteriorly.2 Pintar et al. reported the average length of the ISL in the lumbar spine of 18 studied specimens as 16 mm with a standard deviation of 3.2 mm, and a mean cross-sectional area of 35.1 mm2 with a standard deviation of 15.0 mm2.5
Heylings, in 1978, postulated that in order to allow the ligament to maintain a physiologically desirable degree of tensile control throughout the movement from extension to flexion while still being stable enough to bring this movement to a halt, the ligaments needed to be directed almost radially with respect to the axis of movement, as seen in the collateral ligaments of the joints in the limbs.16 The fibers that cross the interspinous space in a posterocranial direction fulfill these criteria, but if the fibers were running in the posterocaudal direction they would either limit flexion too early, if the fibers ran a straight course between their attachments, or they would be so lax in extension that the ligament would be useless in controlling in the spine except in the movement of flexion.16
Biomechanics
The ISL functions along with the LF and SSL to provide protection and stability to the vertebral joints and to store muscular energy during movement.13 It functions weakly to limit flexion especially in the lumbar spine; the ISL also serves to resist separation of the spinous processes because the fibers run almost perpendicular to the direction of separation of the spinous processes.2,16 Hindle and colleagues found that the supraspinous and interspinous ligaments carried very little load during the first half of flexion but resisted a much greater load towards the end of flexion.19 They suggest that while these ligaments are useful in limiting passive flexion when acting in concert with other support structures, they lack much mechanical function when isolated.19 They also noted that when the SSL was removed, the ISL resisted 75% of the load on its own.19 The ISL is the only spinal ligament that is not loaded during lateral bending.8 When compared to the other lumbar ligaments, this ligament was recorded to exhibit the lowest overall stiffness, at a value of 11.5 +/- 6.6 Nmm-1, with the joint capsule giving the highest stiffness at a value of 33.9 +/- 10.7 Nmm-1 as well as the lowest stress value, ranging between 1.8–5.9 MPa.5
INTERTRANSVERSE LIGAMENT
Anatomy
The intertransverse ligament (ITL) (Fig. 2-1) is a complicated ligament, interpreted in a variety of ways, that consists of sheets of connective tissue that extend from the superior border of one transverse process to the inferior border of the transverse process above.1,2,3,6 In the cervical region, this ligament consists of a few irregular and scattered fibers interspersed and largely replaced by the intertransverse muscles.1,6 The ITL is composed of rounded fibrous cords in the thoracic region intimately blended with adjacent muscles and thin, membranous fibers in the lumbar region.1,3,6 Thus, Bogduk states that the ITL is not a true ligament, but rather a membranous continuation of muscular fibers, with the medial and lateral continuations comprising part of a complex fascial system that separates certain paravertebral compartments.2 In fact, Bogduk states that the only true ligament in the intertransverse area is the ligament of Bourgery; this ligament connects the base of the transverse process to the mammillary process below.2
The ITL is not considered a true ligament, as it lacks distinct medial and lateral borders, and its collagen fibers are not as densely packed nor are they as regularly oriented as the fibers of true ligaments.2,23 An additional reason for why this ligament has been questioned as a true ligament is due to its fibrous fascicles between the transverse processes of the adjacent vertebrate not directly attaching to the upper and lower transverse processes, as Jiang et al. described in 1994 using a study of 32 human cadavers.23 This study suggested that the ligament actually consisted of tendinous fascicles of the semispinalis thoracis muscle and tendons of the levatores costrarum longi muscle, with the former fascicles arising from the transverse process and inserting via tendons into the spinous process and the latter fascicles arising from the ends of the transverse process and passing obliquely downward and laterally until eventually inserting into the outer surface of the rib caudal to the vertebrae from which it originated.23 This hypothesis was corroborated by these fascicular fibers, though thought to be ligamentous fibers, being inseparable from the muscular fibers during dissection of the 32 human cadavers.23 These features suggest that the ITL resembles a membrane rather than a ligament, with its ligamentous appearance produced by the interweaving of muscle tendons between adjacent transverse processes.23
In the lumbar spine, the ITL forms a septum dividing the anterior musculature from the posterior musculature in the intertransverse spaces.2 Laterally, the ligament divides into two layers: an anterior layer of thoracolumbar fascia that covers the front of the quadratus lumborum muscle and a posterior layer that blends with the transverse abdominis aponeurosis to form the middle layer of the thoracolumbar fascia.2 Medially, the ITL splits into dorsal and ventral leaves.2 The dorsal leaf extends medially and attaches to the lateral margin of the lamina of the vertebra lying opposite to the intertransverse space, while inferiorly blending with the capsule of the adjacent facet joint.2 Meanwhile, the ventral leaf curves forward and extends over the lateral surface of vertebral bodies and blends with lateral margins of the ALL.2 By covering the lateral aspect of the vertebral column, the ventral leaf forms a membranous sheet that closes the outer end of the interventricular foramen.2 As such, this ventral leaf has two noted perforations that transmit structures into and out of the IV foramen.2 Running through the superior opening are nerve branches to the psoas muscle, and running through the inferior opening are the ventral rami of spinal nerves, as well as spinal branches of lumbar arteries and veins.2 Sandwiched between the dorsal and ventral leaves is a wedge-shaped space called the superior articular recess that serves to accommodate movements of the sub-adjacent facet joint.2 This recess is filled with fat that is continuous with the intra-articular joint below, via the foramen in the superior capsule.2
Biomechanics
Similar to most of the other ligaments of the subaxial spine, the ITL is loaded during flexion.8 The ITL and ALL carry the highest load during lateral bending movements of the vertebral column.8 The ITL, however, is the only ligament that is not loaded during torsion. Gudavalli and Triano assessed ligament loads using a computer model and concluded that the SSL undergoes the maximum increase in length during torsion, followed by the ISL, LF, facet capsular ligament, and finally, the ITL.18
FACET CAPSULAR LIGAMENT
Anatomy
The facet capsular ligament fully encases the facet joint by covering the joint in a rostral to caudal direction with a non-uniform thickness.24 The lumbar facet capsule has been reported to be approximately 2.0 mm thick in the posterior region while being as much as 3.2 mm thick in the anterior region.24 The superior and inferior regions of the capsule have been reported to be approximately 2.4 mm thick.24 Gray’s Anatomy identifies three distinct types of intracapsular structures: adipose tissue fat pads (anterosuperior, posteroinferior or a combination of the two), fibroadipose meniscoids (located at the superior or inferior pole or at both poles of the capsule), and connective tissue rims (either anterior or posterior or both).1 While the fat pads of the facet capsules are similar to those found in many other joint capsules, the connective tissue rims of the zygapophysial joint are representative of the capsular ligament (CL).1 Each joint is surrounded by a thin, loose CL, which attaches to the margins of the articular processes of adjacent vertebrae.3,6 The average length of the CL in the lumbar spine in 24 individuals’ capsular ligaments was measured to be 16.4 mm (± 2.9) and an average cross-sectional area of 43.8 mm2 (± 28.3).5
Biomechanics
Together with other accessory ligaments of the vertebral column, the capsular ligaments help stabilize the vertebral column and its joints. In the cervical region, the loose nature of this ligament allows for a wide range of movement.6 In the sacral spine, however, the CL functions to prevent the lumbar vertebra from sliding anteriorly down the incline of the sacrum.6 This arises as the facets on S1 face posteromedially and interlock with the anterolateral facing inferior facets of the L5 vertebra.6
Due to the make-up of the facet capsular ligament, it can only provide mechanical resistance when the two adjacent vertebrae articulating in the zygapophysial joint undergo movement relative to one another.24 The role of the ligament was demonstrated in studies using C3-C7 cadaveric spine segments subjected to 100 N of compression with either 2 nanometer (nm) of sagittal bending or 5 nm of axial torsion, before and after graded bilateral removal of the capsular ligament.24 After removal of 50% of the facet capsule, axial rotation increased by 19% when torsion was applied, while the vertical distance between the C4 and C6 spinous processes increased by 5% under flexion.24 The extent of axial torsion and posterior displacement increased by 25% and 32%, respectively, after 75% of the facet capsule was removed.24 The increases in the range of motion observed after capsular transection or removal supports the hypothesis that the facet capsular ligament provides a substantial contribution to constraining vertebral motion, particularly flexion and lateral bending/torsion.24
ILIOLUMBAR LIGAMENT
Anatomy
The iliolumbar ligament (Figs. 2-1 and 2-4) is attached to the tip of anteroinferior aspect of the L5 transverse process bilaterally, as well as the L4 transverse process in some cases, and radiates laterally to attach to the pelvis.1,2 The iliolumbar ligament begins to radiate as it passes laterally from the transverse process and splits into two bands, upper and lower, to attach to the pelvis.1 The lower bands (the lumbosacral portion of the iliolumbar ligament) originate from the inferior aspect of the L5 transverse process and travel to the anterosuperior lateral surface of the sacrum and eventually blend in with the anterior sacroiliac ligament.1 The upper bands traverse laterally and attach to the inner lip of the iliac crest just anterior to the sacroiliac articulation, with the superior portion of the upper bands becoming continuous with the lumbodorsal fascia.1,2 The psoas major muscle surrounds the iliolumbar ligament anteriorly, while the muscles occupying the vertebral groove surround the iliolumbar ligament posteriorly and superiorly by the quadratus lumborum.1
Early anatomists postulated that the iliolumbar ligament consisted of 5 parts.2 The anterior part was a well-developed ligamentous band whose fibers span the entire anteroinferior border of the L5 transverse process (from the border of L5 vertebral body to the tip of the L5 transverse process).2 As the fibers from the medial end of the L5 transverse process pass laterally, they begin to cover the fibers arising from the lateral end and the tip of the L5 transverse process, such that all the fibers become continuous as the band leaves the transverse process and passes posterolaterally to attach to the ilium.2 The upper surface of this anterior bundle of the iliolumbar ligament forms the site of attachment of the ligament to the inferior portion of the quadratus lumborum muscle.2 The superior bundle of the iliolumbar ligament is thought to be formed from the anterior and posterior thickenings of the fascia that surround the base of the quadratus lumborum muscle; these thickenings will attach to the anterosuperior border of the L5 transverse process near the lateral tip.2 As this bundle travels laterally, it separates to pass in front of and behind the quadratus lumborum muscle and ultimately, attach to the ilium superiorly and inferiorly blend with the anterior ligament to form a channel from which the quadratus lumborum muscle arises.2 The posterior bundle of the iliolumbar ligament originates from the tip and posterior border of the L5 transverse process and inserts into the ligamentous area of the ilium behind the origin of the quadratus lumborum; on a unique side note, the deepest fibers of the longissimus lumborum arise from the posterior bundle of the iliolumbar ligament.2 The fourth part of the iliolumbar ligament is the inferior bundle, which arises from the lower border of the transverse process and the body of L5 vertebra.2 These fibers pass downwards and laterally across the surface of the anterior sacroiliac ligament to attach to the superoposterior part of the iliac fossa.2 In order to distinguish these fibers from the anterior sacroiliac ligament, one must observe the oblique orientation of the inferior bundle of the iliolumbar ligament.2 The final portion of the iliolumbar ligament is the vertical bundle, which arises from the anteroinferior border of the L5 transverse process and descends almost vertically to attach to the posterior end of the iliopectineal line of the pelvis.2 The significance of this bundle lies in its formation of the lateral margin of the channel through which the L5 ventral ramus enters the pelvis.2
According to Bogduk, a recent study confirmed the presence of anterior and posterior parts of the iliolumbar ligament but denied the existence of the superior part, while not commenting on the inferior and vertical part.2 This study stated that the superior part is actually the anterior fascia of the quadratus lumborum muscle and as such, lacks the characteristic features of a true ligament that includes the oriented collagen fibers passing directly from one bone to another.2 The vertical and inferior bundles of the iliolumbar ligament were overlooked as part of the ventral sacroiliac ligament because these bundles attach to the lumbar portion and ilium and not to the sacrum and ilium as previously thought; thus, these bundles are now thought to deserve the name “iliolumbar ligament” and not “ventral sacroiliac ligament.”2
In 1998, Hanson et al. found that the mean length of the iliolumbar ligament in Caucasians was approximately 33.2 mm while the length in African Americans was approximately 61.8 mm.25 These differences were also observed to hold true in both sexes, i.e., African American females had iliolumbar ligaments that were longer than those of Caucasian females.25
Thus, the numerous insertions and fiber directions of the iliolumbar ligament suggest a diversity of biomechanical functions for the iliolumbar ligament.26
Biomechanics
The iliolumbar ligament is the most important ligament to restraining excess movement and to maintaining stability at the lumbosacral junction.26,27 The iliolumbar ligament is composed of two bands, an anterior and a posterior band, both of which serve different functions.27 While its overall function is multifocal, the iliolumbar ligament, as a whole, is thought to be a ligament that prevents forward sliding of the L5 vertebrae onto the sacrum.2 The anterior band of the iliolumbar ligament is thought to be responsible for “squaring” the L5 vertebrae on the sacrum and preventing the tilt of the vertebrae in the coronal plane, as well as for preventing lateral bending; conversely, the posterior band is thought to prevent anterior slipping of the L5 vertebrae over the sacrum during weight-bearing movements and to help to resist forward bending.2,27,28 Together with the strong intervertebral disc, the iliolumbar ligament helps stabilize the lumbosacral junction and may play a significant role in maintaining the lumbosacral stability in patients with lumbosacral pathology.2,27
During flexion, the iliolumbar ligament will significantly limit anterior displacement of L5 vertebra.28 When the iliolumbar was divided bilaterally, there was a 77.5% increase noted in anterior flexion of L5, with the posterior bands accounting for the majority, approximately 61.2%, of the increase and the anterior bands contributing only a small amount to the anterior stability of the L5.28 Meanwhile, during extension, a 20.41% increase in flexion of L5 was noted after bilateral division of the ligament; in this case, however, it was the anterior band that contributed to all of the stability during extension.28 Lateral bending with bilateral division of the ligament did result in a dramatic increase of 141.7% in flexion of L5 during contralateral bending, with much of this increase being attributed to the anterior bands.28 During torsion of the L5 vertebrae, division of the iliolumbar ligament did not produce much change in the flexion of L5, but both bands together did restrict torsion by 5.3%.28 These results by Sims and Moorman suggest that the iliolumbar ligament plays a very insignificant role in limiting the displacement of L5 onto the sacrum during torsion, thus further suggesting that other structures are primarily responsible for limiting this displacement during torsion.28 Therefore, Sims and Moorman theorized that the iliolumbar ligament is the probable cause of a majority of chronic low back pain.28
Yamamoto et al. performed in-vitro studies on 4 fresh cadavers of males aged 25–45 (total of 8 ligament specimens) to evaluate the biomechanical function of the iliolumbar ligament, both intact and after transection of the ligament.29 This study involved applying a load of 10 N•m to the intact iliolumbar ligament, a right transected iliolumbar ligament, and bilateral transected iliolumbar ligaments, with the movements of the vertebral column tested before and after the transaction.29 Flexion increased by 0.9o or approximately 12% after removal of right iliolumbar ligament and by 1.7o or approximately 23% after bilateral iliolumbar ligament removal.29 Extension increased by 0.6o (11%) with right iliolumbar ligament transection and by 1.1o (20%) with bilateral iliolumbar ligament transaction.29 Iliolumbar ligaments significantly restricted the motion of the vertebral column to the contralateral side when under torsional load.29 Thus, bilateral iliolumbar ligaments significantly restrict the movements of the lumbosacral junction in the directions of flexion and extension, with a slight increase in destabilization seen with unilateral transection and a statistically significant increase in destabilization seen with bilateral transection.29 However, when comparing all the movements of flexion, extension, right and left lateral bending, and right and left axial rotation, the iliolumbar ligament seems to be most effective in resisting lateral bending of the vertebral column.29
In addition to stabilizing the lumbosacral junction, the iliolumbar ligament may also play an important role in stabilizing the sacroiliac joint by restraining excess movement and thus possibly helping to prevent onset of low back pain.26,30 In his 2003 study, Pool-Goudzwaard defined stability as the ability of a joint to bear loading forces without allowing for any uncontrolled displacements.30 He states that the ligaments of this lumbopelvic and sacroiliac region contribute to stability by controlling the relative positions of the joint, thus restricting the joint to only those positions that the joint can handle when loading forces are applied.30 The existence of the sacroiliac part of the iliolumbar ligament, verified by MRI and cryosectioning of the pelvis in the coronal and transverse planes, reinforces the thinking that the iliolumbar ligament has a direct restraining effect on movements in the sacroiliac joints.26 In 2001, Pool-Goudzwaard et al. studied 12 human specimens and applied incremental moment to the sacroiliac joint to induce rotation in the sagittal plane.26,30 The results of this study indicated that the sacroiliac joint mobility in the sagittal plane increased monumentally after the total transection of both iliolumbar ligaments, with most of the increase seen after cutting the ventral band of the ligament.30 This suggests that the iliolumbar ligaments restrict sacroiliac joint mobility in the sagittal plane with the ventral band of the iliolumbar ligament contributing to most of this restriction.30 Strangely, the locality of the ventral band of the iliolumbar ligament seems to draw skepticism as to how this band is able to restrict the sacroiliac joint’s mobility in the sagittal plane.30 Pool-Goudzwaard, however, offered an explanation in his 2003 study that postulated that this phenomenon could be due to the orientation of the auricular surfaces of the sacroiliac joint with respect to the fiber direction of the ventral band of the iliolumbar ligament.30 The shape of the sacrum is a wedge with the ventral (anterior) aspect of the sacrum being larger than the dorsal (posterior) aspect of the sacrum.30 With the auricular surfaces of sacroiliac joints being oriented dorsomedially and ventrolaterally, one would expect most of the restriction from the ligaments during sagittal rotation of the sacroiliac joint to be parallel to this orientation of the auricular surfaces.30 Since the most ventral fibers of the ventral band of the iliolumbar ligament are arranged more parallel to the auricular surfaces of the sacroiliac joint compared to the fibers of the iliolumbar ligament’s other components, loading of the ventral band of the iliolumbar ligament is likely to be stimulated by sacroiliac rotation in the sagittal plane.30
Several recent studies of the lumbopelvic region have demonstrated support for the hypothesis that loss of the stability of the sacroiliac joints is crucial in the etiology of non-specific low back pain.30 It is now believed, however, that a variety of factors in addition to loss of ligamentous support, such as a decrease in muscle forces and the dysfunction of the mechanoreceptors in the ligamentous system of the lumbosacral area that are important in activating the muscles necessary for posture control, all play a role in the development of non-specific low back pain.30
Lastly, short segmental bands of connective tissue that cross near the intervertebral foramina have been termed the transforaminal ligaments (Fig. 2-6). Although the function of such ligaments is uncertain, they can be space occupying and potentially compress structures entering and leaving the intervertebral foramen (Fig. 2-7).
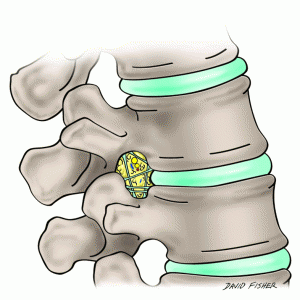
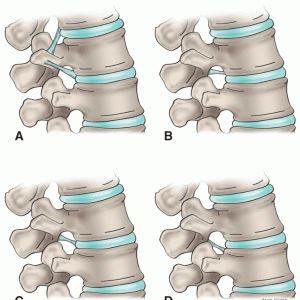
SUGGESTED READINGS
- Alderink GJ. The sacroiliac joint: review of anatomy, mechanics, and function. J Orthop Sports Phys Ther. 1991;13(2):71-84.
- DonTigny RL. Function and pathomechanics of the sacroiliac joint: a review. Phys Ther. 1985;65(1):35-44.
- Goode A, Hegedus EJ, Sizer P, Brismee JM, Linberg A, Cook CE. Three-dimensional movements of the sacroiliac joint: a systematic review of the literature and assessment of clinical utility. J Man Manip Ther. 2008;16(1):25-38.
- Hammer N, Steinke H, Slowik V, Josten C, Stadler J, Böhme J, Spanel-Borowski K. The sacrotuberous and the sacrospinous ligament--a virtual reconstruction. Ann Anat. 2009;191(4):417-425.
- McGrath C, Nicholson H, Hurst P. The long posterior sacroiliac ligament: a histological study of morphological relations in the posterior sacroiliac region. Joint Bone Spine. 2009;76(1):57-62.
- Morris CE. Low Back Syndromes: Integrated Clinical Management. New York: McGraw-Hill; 2005.
- Onan OA, Heggeness MH, Hipp JA. A motion analysis of the cervical facet joint. Spine. 1998;23(4):430-439.
- Serhan HA, Varnavas G, Dooris AP, Patwardhan A, Tzermiadianos M. Biomechanics of the posterior lumbar articulating elements. Neurosurg Focus. 2007;22(1):E1.
- Sturesson B, Selvik G, Udén A. Movements of the sacroiliac joints. A roentgen stereophotogrammetric analysis. Spine. 1989;14(2):162-165.
- Vleeming A, Stoeckart R, Snijders CJ. The sacrotuberous ligament: a conceptual approach to its dynamic role in stabilizing the sacroiliac joint. Clin Biomech. 1989;4(4):201-203.
- Vleeming A, Van Wingerden JP, Snijders CJ, Stoeckart R, Stijnen T. Load application to the sacrotuberous ligament; influences on sacroiliac joint mechanics. Clin Biomech. 1989;4(4):204-209.
- Wilder DG, Pope MH, Frymoyer JW. The functional topography of the sacroiliac joint. Spine. 1980;5(6):575-579.
- Willard FH, Carreiro JE, Manko W. The long posterior interosseous ligament and the sacrococcygeal plexus. In: Proceedings of the Third Interdisciplinary World Congress on Low Back and Pelvic Pain. Vienna, Austria: World Congress of Low Back Pain and Pelvic Pain; 1998:207-209.
REFERENCES
- Bannister LH, Berry MM, Collins P, Dyson M, Dussek JE, Ferguson MWJ, eds. Gray’s Anatomy. 38th ed. New York: Churchill Livingston; 1996.
- Bogduk N. Clinical Anatomy of the Lumbar Spine and Sacrum. 3rd ed. New York: Churchill Livingston; 1997.
- Dickman CA, Rosenthal DJ, Perin NI. Thoracoscopic Spine Surgery. New York: Thieme Medical Publishers; 1999.
- Lang J. Clinical Anatomy of the Cervical Spine. New York: Thieme Medical Publishers; 1993.
- Pintar FA, Yoganandan N, Myers T, Elhagediab A, Sances A Jr. Biomechanical properties of human lumbar spine ligaments. J Biomech. 1992;25(11):1351-1356.
- Moore KL, Dalley AF, Agur AMR. Clinically Oriented Anatomy. 6th ed. Baltimore: Lippincott Williams & Wilkins; 2010.
- Neumann P, Keller TS, Ekström L, Perry L, Hansson TH, Spengler DM. Mechanical properties of the human lumbar anterior longitudinal ligament. J Biomech. 1992;25(10):1185-1194.
- Zander T, Rohlmann A, Bergmann G. Influence of ligament stiffness on the mechanical behavior of a functional spinal unit. J Biomech. 2004;37(7):1107-1111.
- Neumann P, Keller T, Ekström L, Hult E, Hansson T. Structural properties of the anterior longitudinal ligament. Correlation with lumbar bone mineral content. Spine. 1993;18(5):637-645.
- Panjabi MM, Goel VK, Takata K. Physiologic strains in the lumbar spinal ligaments. An in vitro biomechanical study 1981 Volvo Award in Biomechanics. Spine. 1982;7(3):192-203.
- Chazal J, Tanguy A, Bourges M, Gaurel G, Escande G, Guillot M, Vanneuville G. Biomechanical properties of spinal ligaments and a histological study of the supraspinal ligament in traction. J Biomech. 1985;18(3):167-176.
- Yong-Hing K, Reilly J, Kirkaldy-Willis WH. The ligamentum flavum. Spine. 1976;1(4):226-234.
- Yahia LH, Garzon S, Strykowski H, Rivard CH. Ultrastructure of the human interspinous ligament and ligamentum flavum. A preliminary study. Spine. 1990;15(4):262-268.
- Panjabi MM, Greenstein G, Duranceau J, Nolte LP. Three-dimensional quantitative morphology of lumbar spinal ligaments. J Spinal Disord. 1991;4(l):54-62.
- Nachemson AL, Evans JH. Some mechanical properties of the third human lumbar interlaminar ligament (Iigamentum flavum). J Biomech. 1968;1(3):211-220.
- Heylings DJ. Supraspinous and interspinous ligaments of the human lumbar spine. J Anat. 1978;125(1):127-131.
- Parke WW. The Spine, vol. 1. Philadelphia, London, Toronto: W. B. Saunders Co; 1975.
- Gudavalli MR, Triano JJ. An analytical model of lumbar motion segment in flexion. J Manipulative Physiol Ther. 1999;22(4):201-208.
- Hindle RJ, Pearcy MJ, Cross A. Mechanical function of the human lumbar interspinous and supraspinous ligaments. J Biomed Eng. 1990;12(4):340-344.
- Spalteholz W. Hand Atlas of Human Anatomy. 7th ed, vol. 1. Philadelphia, London: JB Lippincott Co; 1943.
- Agur AMR, Dalley AF. Grant’s Atlas of Anatomy. 11th ed. Baltimore: Lippincott Williams and Wilkins; 2005.
- Rissanen, PM. The surgical anatomy and pathology of the supraspinous and interspinous ligaments of the lumbar spine with special reference to ligament ruptures. Acta Orthop Scand Suppl. 1960;46:1-100.
- Jiang H, Raso JV, Moreau MJ, Russell G, Hill DL, Bagnall KM. Quantitative morphology of the lateral ligaments of the spine: assessment of their importance in maintaining lateral stability. Spine. 1994;19(23):2676-2682.
- Jaumard NV, Welch WC, Winkelstein BA. Spinal facet joint biomechanics and mechanotransduction in normal, injury and degenerative conditions. J Biomech Eng. 2011;133(7):071010.
- Hanson P, Magnusson SP, Sorensen H, Simonsen EB. Differences in the iliolumbar ligament and the transverse process of the L5 vertebra in young white and black people. Acta Anat. 1998;163(4):218-223.
- Pool-Goudzwaard AL, Kleinrensink GJ, Snijders CJ, Entius C, Stoeckart R. The sacroiliac part of the iliolumbar ligament. J Anat. 2001;199(Pt 4):457-463.
- Luk KD, Ho HC, Leong JC. The iliolumbar ligament: a study of its anatomy, development, and clinical significance. J Bone Joint Surg Br. 1986;68(2):197-200.
- Sims JA, Moorman SJ. The role of the iliolumbar ligament in low back pain. Med Hypotheses. 1996;46(6):511-515.
- Yamamoto I, Panjabi MM, Oxland TR, Crisco JJ. The role of the iliolumbar ligament in the lumbosacral junction. Spine. 1990;15(11):1138-1141.
- Pool-Goudzwaard AL, Hoek van Dijke G, Mulder P, Spoor C, Snijders CJ, Stoeckart R. The iliolumbar ligament: its influence on stability of the sacroiliac joint. Clin Biomech. 2003;18(2):99-105.